3.1 Introduction
The concentration of CO2 in the atmosphere has risen from close
to 280 parts per million (ppm) in 1800, at first slowly and then progressively
faster to a value of 367 ppm in 1999, echoing the increasing pace of global
agricultural and industrial development. This is known from numerous, well-replicated
measurements of the composition of air bubbles trapped in Antarctic ice. Atmospheric
CO2 concentrations have been measured directly with high precision
since 1957; these measurements agree with ice-core measurements, and show a
continuation of the increasing trend up to the present.
Several additional lines of evidence confirm that the recent and continuing
increase of atmospheric CO2 content is caused by anthropogenic CO2
emissions – most importantly fossil fuel burning. First, atmospheric O2
is declining at a rate comparable with fossil fuel emissions of CO2
(combustion consumes O2). Second, the characteristic isotopic signatures
of fossil fuel (its lack of 14C, and depleted content of 13C)
leave their mark in the atmosphere. Third, the increase in observed CO2
concentration has been faster in the northern hemisphere, where most fossil
fuel burning occurs.
Atmospheric CO2 is, however, increasing only at about half the rate
of fossil fuel emissions; the rest of the CO2 emitted either dissolves
in sea water and mixes into the deep ocean, or is taken up by terrestrial ecosystems.
Uptake by terrestrial ecosystems is due to an excess of primary production (photosynthesis)
over respiration and other oxidative processes (decomposition or combustion
of organic material). Terrestrial systems are also an anthropogenic source of
CO2 when land-use changes (particularly deforestation) lead to loss
of carbon from plants and soils. Nonetheless, the global balance in terrestrial
systems is currently a net uptake of CO2.
The part of fossil fuel CO2 that is taken up by the ocean and the
part that is taken up by the land can be calculated from the changes in atmospheric
CO2 and O2 content because terrestrial processes of CO2
exchange involve exchange of oxygen whereas dissolution in the ocean does not.
Global carbon budgets based on CO2 and O2 measurements
for the 1980s and 1990s are shown in Table 3.1. The human
influence on the fluxes of carbon among the three “reservoirs” (atmosphere,
ocean, and terrestrial biosphere) represent a small but significant perturbation
of a huge global cycle (Figure 3.1).
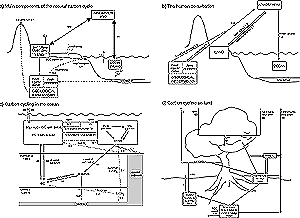
Figure 3.1: The global carbon cycle: storages (PgC) and fluxes
(PgC/yr) estimated for the 1980s. (a) Main components of the natural cycle.
The thick arrows denote the most important fluxes from the point of view
of the contemporary CO2 balance of the atmosphere: gross primary
production and respiration by the land biosphere, and physical air-sea
exchange. These fluxes are approximately balanced each year, but imbalances
can affect atmospheric CO2 concentration significantly over
years to centuries. The thin arrows denote additional natural fluxes (dashed
lines for fluxes of carbon as CaCO3), which are important on longer time-scales.
The flux of 0.4 PgC/yr from atmospheric CO2 via plants to inert
soil carbon is approximately balanced on a time-scale of several millenia
by export of dissolved organic carbon (DOC) in rivers (Schlesinger, 1990).
A further 0.4 PgC/yr flux of dissolved inorganic carbon (DIC) is derived
from the weathering of CaCO3, which takes up CO2 from the atmosphere
in a 1:1 ratio. These fluxes of DOC and DIC together comprise the river
transport of 0.8 PgC/yr. In the ocean, the DOC from rivers is respired
and released to the atmosphere, while CaCO3 production by marine organisms
results in half of the DIC from rivers being returned to the atmosphere
and half being buried in deep-sea sediments - which are the precursor
of carbonate rocks. Also shown are processes with even longer time-scales:
burial of organic matter as fossil organic carbon (including fossil fuels),
and outgassing of CO2 through tectonic processes (vulcanism).
Emissions due to vulcanism are estimated as 0.02 to 0.05 PgC/yr (Williams
et al., 1992; Bickle, 1994). (b) The human perturbation (data from Table
3.1). Fossil fuel burning and land-use change are the main anthropogenic
processes that release CO2 to the atmosphere. Only a part of
this CO2 stays in the atmosphere; the rest is taken up by the
land (plants and soil) or by the ocean. These uptake components represent
imbalances in the large natural two-way fluxes between atmosphere and
ocean and between atmosphere and land. (c) Carbon cycling in the ocean.
CO2 that dissolves in the ocean is found in three main forms
(CO2, CO32-, HCO3-, the sum of which is DIC). DIC is transported
in the ocean by physical and biological processes. Gross primary production
(GPP) is the total amount of organic carbon produced by photosynthesis
(estimate from Bender et al., 1994); net primary production (NPP) is what
is what remains after autotrophic respiration, i.e., respiration by photosynthetic
organisms (estimate from Falkowski et al., 1998). Sinking of DOC and particulate
organic matter (POC) of biological origin results in a downward flux known
as export production (estimate from Schlitzer, 2000). This organic matter
is tranported and respired by non-photosynthetic organisms (heterotrophic
respiration) and ultimately upwelled and returned to the atmosphere. Only
a tiny fraction is buried in deep-sea sediments. Export of CaCO3 to the
deep ocean is a smaller flux than total export production (0.4 PgC/yr)
but about half of this carbon is buried as CaCO3 in sediments; the other
half is dissolved at depth, and joins the pool of DIC (Milliman, 1993).
Also shown are approximate fluxes for the shorter-term burial of organic
carbon and CaCO3 in coastal sediments and the re-dissolution of a part
of the buried CaCO3 from these sediments. (d) Carbon cycling on land.
By contrast with the ocean, most carbon cycling through the land takes
place locally within ecosystems. About half of GPP is respired by plants.
The remainer (NPP) is approximately balanced by heterotrophic respiration
with a smaller component of direct oxidation in fires (combustion). Through
senescence of plant tissues, most of NPP joins the detritus pool; some
detritus decomposes (i.e., is respired and returned to the atmosphere
as CO2) quickly while some is converted to modified soil carbon,
which decomposes more slowly. The small fraction of modified soil carbon
that is further converted to compounds resistant to decomposition, and
the small amount of black carbon produced in fires, constitute the “inert”
carbon pool. It is likely that biological processes also consume much
of the “inert” carbon as well but little is currently known
about these processes. Estimates for soil carbon amounts are from Batjes
(1996) and partitioning from Schimel et al. (1994) and Falloon et al.
(1998). The estimate for the combustion flux is from Scholes and Andreae
(2000). t’ denotes the turnover time for different components
of soil organic matter.
|
This chapter summarises current knowledge of the global carbon cycle, with
special reference to the fate of fossil fuel CO2 and the factors
that influence the uptake or release of CO2 by the oceans and land.
These factors include atmospheric CO2 concentration itself, the naturally
variable climate, likely climate changes caused by increasing CO2
and other greenhouse gases, changes in ocean circulation and biology, fertilising
effects of atmospheric CO2 and nitrogen deposition, and direct human
actions such as land conversion (from native vegetation to agriculture and vice
versa), fire suppression and land management for carbon storage as provided
for by the Kyoto Protocol (IPCC, 2000a). Any changes in the function of either
the terrestrial biosphere or the ocean - whether intended or not - could potentially
have significant effects, manifested within years to decades, on the fraction
of fossil fuel CO2 that stays in the atmosphere. This perspective
has driven a great deal of research during the years since the IPCC WGI Second
Assessment report (IPCC, 1996) (hereafter SAR) (Schimel et al., 1996; Melillo
et al., 1996; Denman et al., 1996). Some major areas where advances have been
made since the SAR are as follows:
- Observational research (atmospheric, marine and terrestrial) aimed at a
better quantification of carbon fluxes on local, regional and global scales.
For example, improved precision and repeatability in atmospheric CO2
and stable isotope measurements; the development of highly precise methods
to measure changes in atmospheric O2 concentrations; local terrestrial
CO2 flux measurements from towers, which are now being performed
continuously in many terrestrial ecosystems; satellite observations of global
land cover and change; and enhanced monitoring of geographical, seasonal and
interannual variations of biogeochemical parameters in the sea, including
measurements of the partial pressure of CO2 (pCO2)
in surface waters.
- Experimental manipulations, for example: laboratory and greenhouse experiments
with raised and lowered CO2 concentrations; field experiments on
ecosystems using free-air carbon dioxide enrichment (FACE) and open-top chamber
studies of raised CO2 effects, studies of soil warming and nutrient
enrichment effects; and in situ fertilisation experiments on marine ecosystems
and associated pCO2 measurements.
- Theory and modelling, especially applications of atmospheric transport models
to link atmospheric observations to surface fluxes (inverse modelling); the
development of process-based models of terrestrial and marine carbon cycling
and programmes to compare and test these models against observations; and
the use of such models to project climate feedbacks on the uptake of CO2
by the oceans and land.
As a result of this research, there is now a more firmly based knowledge of
several central features of the carbon cycle. For example:
- Time series of atmospheric CO2, O2 and 13CO2
measurements have made it possible to observationally constrain the partitioning
of CO2 between terrestrial and oceanic uptake and to confirm earlier
budgets, which were partly based on model results.
- In situ experiments have explored the nature and extent of CO2
responses in a variety of terrestrial ecosystems (including forests), and
have confirmed the existence of iron limitations on marine productivity.
- Process-based models of terrestrial and marine biogeochemical processes
have been used to represent a complex array of feedbacks in the carbon cycle,
allowing the net effects of these processes to be estimated for the recent
past and for future scenarios.
Table 3.1: Global CO2 budgets (in PgC/yr)
based on intra-decadal trends in atmospheric CO2 and O2.
Positive values are fluxes to the atmosphere; negative values represent
uptake from the atmosphere. The fossil fuel emissions term for the 1980s
(Marland et al., 2000) has been slightly revised downward since the SAR.
Error bars denote uncertainty (± 1s), not interannual variability,
which is substantially greater. |
 |
|
1980s
|
1990s
|
 |
Atmosphere increase
|
3.3 ± 0.1
|
3.2 ± 0.1
|
Emissons (fossil fuel, cement)
|
5.4 ± 0.3
|
6.3 ± 0.4
|
Ocean-atmosphere flux
|
-1.9 ± 0.6
|
-1.7 ± 0.5
|
Land atmsphere fluux* |
-0.2±0.7
|
-.1.4±0.7
|
*partitioned as follows
|
|
|
Land use change
|
1.7 (0.6 to 2.5)
|
NA
|
Residual terrestrial sink
|
-1.9 (-3.8 to 0.3)
|
NA
|
 |
|