3.4.6 Technological and Economic Potential
This section addresses the technological potential to cost-effectively increase
energy efficiency in transport and thereby reduce GHG emissions. Most studies
concentrate on light-duty vehicles because of their 50% share of energy use
and GHG emissions, and on technology or fuel pricing policies. Technical efficiency
improvements, in the absence of complementary fiscal policies, are subject to
a rebound effect in that they reduce the fuel cost of travel. Rebound
effects in the USA amount to about 20% of the potential GHG reductions (Greene,
1999). In Europe, where fuel prices are higher, rebound effects may be as large
as 40% (Michaelis, 1997). Most assessments take the rebound effect into account
when estimating technical efficiency impacts. Fewer studies address policies
such as land use planning, investment in or subsidy of particular transport
modes, or information.
An Asian four-country study of the technological and economic potential to
reduce GHG emissions considered five types of options for GHG mitigation in
transport: (1) improving fuel efficiency, (2) improving transportation system
efficiency, (3) behavioural change, (4) modal split changes, and (5) technological
change (Bose, 1999b). The Indian study concluded that abatement costs for transport
were high relative to options available in other sectors, and projected little
change in transport for emissions constraints less than a 20% reduction from
the baseline. The Bangladesh study, using a different methodology, concluded
that a wide array of near-term technology options had no net cost, but that
the cost of 4-stroke engines for 3-wheeled vehicles fell between US$48 and US$334/tC
reduced, depending on the application. The Thailand study found that lean-burn
engines would improve efficiency by 20% at a negative net cost of US$509/tC.
The Korean study also concluded that several no regrets options
were available, including use of continuously variable transmissions, lean-burn
engines, and exclusive bus lanes.
Recognizing that transportation energy consumption and CO2 emissions increased
by 16% from 1990 to 1995, and that carbon emissions may be 40% higher in 2010
than in 1990 if measures are not taken, the government of Japan has strengthened
energy efficiency standards based on a Front Runners approach, which
sets standards to meet or exceed the highest energy efficiency achieved among
products currently commercialized (MITI/ANRE, 1999). These require a 22.8% improvement
over 1995 new gasoline car fuel economy in 1/km by 2010, and a 13.2% improvement
for gasoline light-duty freight vehicles (Minato, 1998). For diesel-fuelled
vehicles the corresponding requirements are 14.9% and 6.5% by 2005. Technological
improvements in other modes are expected to produce efficiency improvements
of 7% for railways, 3% for ships, and 7% for airlines over the same period (Minato,
1998). Cost-effective technical potentials have also been reported by Kashiwagi
et al. (1999), who cite 27.7 PJ of energy savings in Japans transport
sector achievable at US$0.044/kWh, or less.
There are significant barriers to the kinds of fuel economy improvements described
above, and substantial policy initiatives will be needed to overcome them. In
Europe, for example, the European automobile manufacturers association,
ACEA, and the European Union have agreed to voluntary standards to reduce carbon
emissions from new passenger cars by 25% over the next 10 years. The European
standards will require reducing average fuel consumption of new cars from 7.7
to 5.8 l/100 km, creating a strong incentive to adopt advanced fuel economy
technologies. A survey of 28 European countries identified 334 separate measures
countries were taking to reduce CO2 emissions from transport (Perkins, 1998).
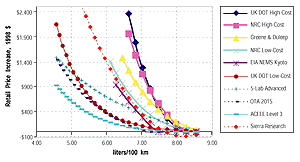
Figure 3.10: Passenger car fuel economy cost curves.
|
At least nine recent studies have assessed the economic potential for technology
to improve light-duty vehicle fuel economy (Weiss et al., 2000; Greene and DeCicco,
1999; Michaelis, 1997). The conclusions of eight of the studies are summarized
in the form of quadratic fuel economy cost curves describing incremental purchase
cost versus the improvement in fuel economy over a typical 8.4 l/100 km passenger
car (Figure 3.10). Most of the technology potential curves reflect a short-run
perspective, considering what can be achieved using only proven technologies
over a 10-year period. The two most pessimistic (which reflect a 1990 industry
view of short-term technology potential) indicate that even a reduction from
8.4 to 6.5 l/100 km would cost nearly US$2000. The curves labelled ACEEE
Level 3 and UK DOT Low-Cost are limited to proven technologies,
but allow substantial trade-offs in performance, transmission-management and
other features that may affect customer satisfaction. The curves labelled 5-lab
and OTA 2015 include the benefits of technologies in development,
but not yet commercialized (NRC, 1992; DeCicco and Ross, 1993; US DOE/EIA, 1998).
The most optimistic of these suggest that an improvement to less than 5.9 l/100
km is possible at an incremental cost of less than US$1000 per vehicle (1998
US$). The Sierra Research (Austin et al., 1999) curve is intended to pertain
to the year 2020, but reflects industry views about technology performance,
and excludes certain key technologies such as hybrids and fuel cell vehicles
that could have dramatic impacts over the next 20 years.
Three of the studies (OTA, 1995b; DeCicco and Ross, 1993; National Laboratory
Directors, 1997) considered more advanced technologies such as those described
above (e.g., direct-injection engines, aluminium-intensive designs, hybrid vehicles,
fuel cells). These concluded that by 2015, consumption rates below 4.7 l/100
km could be attained at costs ranging from under US$1000 to US$1500 per vehicle.
These long-run curves span a range similar to fuel consumption/cost curves for
European passenger cars reported by Denis and Koopman (1998, Figure 3), except
that the base fuel consumption rate is 7 l/100 km as opposed to 8.5 in the USA,
and improvements to the range of 4 to 5 l/100 km were judged achievable at incremental
costs of 2000 to 700 ECU, respectively (1990 ECU).
A lifecycle analysis of the greenhouse gas impacts of nine hybrid electric
and fuel cell vehicles was compared to a 1996 vehicle and an evolved 2020
baseline vehicle for the year 2020 by Weiss et al. (2000). The study concluded
that a hybrid vehicle fuelled by compressed natural gas could reduce GHG emissions
by almost two-thirds relative to the 1996 reference vehicle, and by 50% compared
with an advanced 2020 internal combustion engine vehicle. Other technologies
capable of 50%, or greater lifecycle GHG reductions versus the 1996 reference
vehicle included: gasoline and diesel hybrids, battery-electric, and hydrogen
fuel cell vehicles.
A recent study by five of the US Department of Energys (DOEs) National
Laboratories (Interlaboratory Working Group, 1997) assessed the economic market
potential for carbon reductions, using the EIAs National Energy Modelling
System. Transport carbon emissions were projected to rise from 487 MtC in 1997
to 616 MtC by 2010 in the baseline case. In comparison to the baseline case,
use of cost-effective technologies reduced carbon emissions by 12% in 2010 in
an Efficiency case (Table 3.13). More optimistic
assumptions about the success of R&D produced a reduction of 17% by 2010.
The authors noted that lead times for cost-effectively expanding manufacturing
capacity for new technologies and the normal turnover of the stock of transport
equipment significantly limited what could be achieved by 2010. Efficiency improvements
in 2010 for new transportation equipment were substantially greater (Table
3.14). New passenger car efficiency increased by 36% in the Efficiency
case and by 57% in the more optimistic case (Brown et al., 1998).
Table 3.13: Estimated technological
potential for carbon emissions reductions in the US transportation sector
(Brown et al., 1998). |
 |
|
1990
|
2010
|
2020
|
2030
|
 |
Business as usual (MtC) |
432
|
598
|
665
|
741
|
Technology potential (%) |
|
712
|
1517
|
2740
|
 |
|
1990
|
2010
|
|
|
Baseline
|
Efficiency
|
High efficiencya
|
 |
Transport emissions (MtC) |
432
|
616
|
543
|
513
|
Reduction (%) |
|
|
12
|
17
|
 |
|
Table 3.14: Projected transportation
efficiencies of 5-Laboratory Study
(Interlaboratory Working Group, 1997). |
 |
|
|
2010
|
Determinants |
1997
|
Baseline
|
Efficiency
|
HE/LCa
|
 |
New passenger car l/100 km |
8.6
|
8.5
|
6.3
|
5.5
|
New light truck l/100 km |
11.5
|
11.4
|
8.7
|
7.6
|
Light-duty fleet l/100 kmb |
12.0
|
12.1
|
10.9
|
10.1
|
Aircraft efficiency (seat-l/100 km) |
4.5
|
4.0
|
3.8
|
3.6
|
Freight truck fleet l/100 km |
42.0
|
39.2
|
34.6
|
33.6
|
Rail efficiency (tonne-km/MJ) |
4.2
|
4.6
|
5.5
|
6.2
|
 |
|
Eleven of the US DOEs National Laboratories completed a comprehensive
assessment of the technological potential to reduce GHG emissions from all sectors
of the US economy (National Laboratory Directors, 1997). This study intentionally
made optimistic assumptions about R&D success, and did not explicitly consider
costs or other market factors. The study concluded that the technological potential
for carbon emissions reductions from the US transport sector was 4070
million metric tons of carbon (MtC) by 2010, 100180MtC by 2020 and 200300MtC
by 2030. These compare to total US transportation carbon emissions of 473MtC
in 1997 (note that this base year estimate differs from that for the Interlaboratory
Working Group). The report suggested the following technological potentials
for carbon emissions reductions by mode of transport over the next 25 years:
(1) light-duty vehicles with fuel cells, 50%100%; (2) heavy trucks via
fuel economy improvements, 20%33%; and (3) air transport, 50%. It is difficult
to interpret the practical implications of these conclusions, however, since
no attempt was made by this study to estimate achievable market potentials.
Three European studies of the technical-economic potential for energy savings
and CO2 reduction were reviewed by van Wee and Annema (1999). Generally, the
studies focused on technological options, such as improving the fuel efficiencies
of conventional cars and trucks, promotion of hybrid vehicles, switching trucks
and buses to natural gas, and electrifying buses, delivery trucks, and mopeds.
Only the study for Hanover included investment in improved public transport
as a major policy option. The results, summarized in Table 3.15, suggest that
emissions reductions of 8% to as much as 42% over business-as-usual projections
may be possible.
The effects of a variety of fiscal and regulatory policies on CO2 emissions
from road passenger vehicles have been estimated for Europe over a 15-year forecast
horizon (Jansen and Denis, 1999; Denis and Koopman, 1998). These studies, both
using the EUCARS model developed for the European Commission, concluded that
CO2 reductions on the order of 15% over a baseline case could be achieved in
the 2011 to 2015 time period at essentially zero welfare loss. Among the more
effective policies were fuel taxes based on carbon content, fuel consumption
standards requiring proportional increases for all cars, and the combination
of fuel-consumption based vehicle sales taxes with a fuel tax. When reductions
in external costs and the benefit of raising public revenues are included in
the calculation of social welfare impacts, the feebate (a policy combining subsidies
for fuel efficient vehicles and taxes on inefficient ones) and fuel tax policy
combination was able to achieve CO2 reductions of 20% to 25% in the 2011 to
2015 time period at zero social cost (Jansen and Denis, 1999).
Table 3.15: Assumptions and results
of three European studies |
 |
|
Dutch
|
Hanover
|
EU
|
 |
Base and target years
(length of scenario in years) |
1995, 2020 (25 years)
|
1990, 2010 (20 years)
|
1990, 2000 (10 years)
|
CO2 emissions in target year:
baseline (Mt) |
36.643.3 |
1.9 |
649.8 |
Annual percentage growth in baseline emissions (Mt) |
0.4% to 1.4% per year |
0.6% per year |
1.7% per year |
Solution scenario |
(I) Best technical means,
(II) Intensifying current policy,
(III) Non-conventional local
transport technologies |
(A) Local/regional,
(B) National |
(R) Reasonable restrictive,
(T) Target orientation |
Base and target years
(length of scenario, in years) |
1995, 2020 (25 years) |
1990, 2010 (20 years) |
1990, 2000 (10 years) |
CO2 emission reduction
(transport sector - Mt) |
(I) 1113,
(II) 311,
(III) 18 |
(A) 0.16 and (B) 0.34 |
(R) 84 and (T) 177 |
Reduction of total transport emissions (including non-road
transport) relative to baseline in target year |
(I) 30%,
(II) 8%25%,
(III) 42% |
(A) 8% and (B) 18% |
(R) 13% and (T) 25% |
Economic evaluation
Net annual costs |
Not quantified, though asserted to be <€0 /tC |
Not quantified |
Not quantified |
 |
|