4.3.3. Model Results for Supersonic Aircraft Emissions
4.3.3.1. NOy and H2O Enhancement
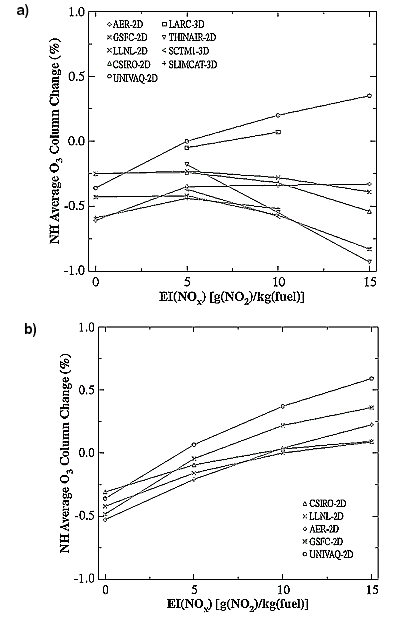
Figure 4-7: Northern Hemisphere total O3 column change as a function of
EI(NOx) in 2015 for an HSCT fleet size of 500 active aircraft with (a) SA0 sulfate distribution
and (b) 4xSA0 sulfate distribution with no sulfur aircraft emissions.
|
Supersonic aircraft emissions of NOx and H2O were incorporated within six 2-D
models and three 3-D models. These supersonic aircraft emissions are primarily
deposited within the Northern Hemisphere LS. Figure
4-6 shows calculated perturbations in NOy and H2O from HSCT emissions in
June 2015 with EI(NOx)=5 (scenario S1c-D). Maximum perturbations occur in the
Northern Hemisphere LS and range from 0.6 to 1.0 ppbv for NOy and from 0.4 to
0.7 ppmv for H2O. Based on the calculated perturbation in NOy and H2O, one can
conclude that the transport fields of these multi-dimensional assessment models
are significantly different. For example, the GSFC model shows a significantly
larger calculated perturbation in Northern Hemisphere lower stratospheric NOy
than the other models (20-40% higher peak NOy abundance). This model also transports
more NOy and H2O higher into the middle stratosphere (MS) and into the Southern
Hemisphere than the other models. By contrast, the AER model tends to isolate
supersonic emission increases in NOy and H2O within the Northern Hemisphere
LS. The THINAIR and LLNL models calculate a NOy perturbation from supersonic
aircraft in the Northern Hemisphere LS that approaches that of the GSFC model.
However, these models transport much less aircraft-enhanced NOy and H2O to the
Southern Hemisphere. CSIRO model NOy and H2O perturbations are similar to those
of LLNL, except that the maximum NOy abundance increase in the Northern Hemisphere
LS is significantly less. The SLIMCAT model shows greater NOy and H2O enhancements
in the Southern Hemisphere than the GSFC model but smaller Northern Hemisphere
perturbations. Aircraft-induced NOy enhancements in the middle stratosphere
are greatest in the THINAIR, GSFC, and SLIMCAT models for Northern Hemisphere
and tropical regions. Overall, the AER, CSIRO, LLNL, and THINAIR models show
the greatest Northern Hemisphere isolation of supersonic aircraft effluent among
the participating assessment models. The UNIVAQ, LARC, and SCTM1 models derive
a maximum Northern Hemisphere LS NOy abundance increase similar to CSIRO. However,
these models derive relatively small horizontal gradients in the LS horizontal
spread in NOy and H2O, extending from Northern Hemisphere mid-latitudes to Southern
Hemisphere high latitudes, suggesting a weak tropical/mid-latitude barrier to
mixing. The UNIVAQ, SLIMCAT, SCTM1, and LARC models generate Southern Hemisphere
LS NOy and H2O magnitudes that are similar to those of the GSFC model. To first
order, the spread in model-derived changes in total O3 for the ensemble of scenarios
shown in Tables 4-11 and 4-12
is a direct result of large differences between supersonic aircraft-induced
perturbations in the NOy and H2O fields.
4.3.3.2. Profile and Column Ozone Change
The percentage change in profile O3 for June is shown in Figure
4-6c for scenario S1c-D. Above 25 km, in all models, local O3 abundance
is reduced as a result of including supersonic aircraft emissions of NOx and
H2O. This result is not surprising because all assessment models represent the
NOx (middle stratosphere) and HOx (upper stratosphere) chemical families as
prime contributors to odd-oxygen loss above 25 km, in approximately the same
relative importance. Therefore, sensitivity to NOx and H2O emissions from supersonic
aircraft exhaust is similar in these regions and roughly proportional to calculated
perturbations in NOy and H2O. Below 25 km, the relative odd-oxygen loss partitioning
between NOx, HOx, and ClOx-BrOx chemical families is highly model-dependent.
This situation is evident in the calculated local O3 change. Models that are
more HOx dominant in the LS tend to show a more positive response to added aircraft
NOx. The opposite is true for models that are more NOx dominant. These variations
are a result of different methodologies of transport and chemistry incorporated
in the individual models.
The percentage change in total column O3 is shown in Figure
4-6d for scenario S1c-D. Six of the models (AER, CSIRO, GSFC, LLNL, SCTM1,
and UNIVAQ) indicate smaller depletions in the tropics and larger depletions
at mid- and high latitudes. The other three models (LARC, SLIMCAT, and THINAIR)
show more complicated features. The LARC model predicts increases in Northern
Hemisphere mid- and high latitudes and decreases in the tropics. The SLIMCAT
model predicts decreases of more than 0.6% in the tropics, with less depletion
predicted at Northern Hemisphere mid- and high latitudes and Southern Hemisphere
mid-latitudes. The THINAIR model generally predicts larger depletions at lower
latitudes. Decreases in Northern Hemisphere polar latitudes of greater than
1% are shown in the AER model results. The GSFC and SLIMCAT models predict decreases
in the Southern Hemisphere polar spring of greater than 1%.
4.3.3.3. Column Ozone Sensitivity to Supersonic Emission
of NOx and H2O
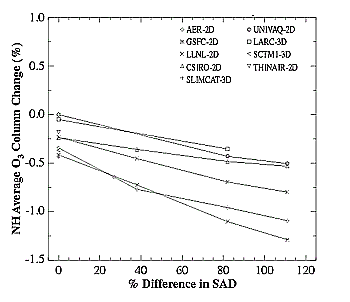
Figure 4-8: Northern Hemisphere total O3 column change as a
function of surface area density (SAD) between 14-21 km and 33-90°N. Calculations
are for EI(NOx)=5 and an HSCT fleet size of 500 aircraft in the 2015 atmosphere.
The 27, 38, 82, and 111% change in SAD correspond to SA7, SA5, SA1, and SA3 as listed
in Table 4-9, respectively.
|
Sensitivity studies of supersonic engine emissions of H2O and NOx were carried
out. These studies investigated individual and combined impacts on O3 from H2O
and NOx emissions within an atmosphere that was volcanically clean (SA0). In
Figure 4-7a, the change in annual average Northern
Hemisphere total column O3 as a function of EI(NOx) is shown for a fleet of
500 supersonic aircraft in the year 2015 (Scenarios S1b-e). The reference atmosphere
included emissions from a representative 2015 fleet of subsonic aircraft. Under
the conditions of these scenarios, the models predict that water emissions [corresponding
to EI(NOx)=0] have the most significant impact. For EI(NOx)=0, the participating
models all derive a total O3 depletion in the 0.2-0.6% range.
For low emission indices of NOx [EI(NOx)=5 to 10], the predicted decrease in
O3 is dominated by emitted H2O; in most models, the addition of NOx emissions
leads to less predicted O3 depletion. Because O3 loss in the lower stratosphere
tends to be dominated by HOx constituents and supersonic aircraft emissions
are most important in the lower stratosphere (see Figure
4-6), the predicted importance of H2O emissions is understandable.
Several models show relatively little sensitivity to NOx emissions over the
EI(NOx)=5 to 15 range. Including NOx emission at an EI(NOx)=5 level, S1c had
little effect on total column O3 change for the LLNL, GSFC, and CSIRO models
[relative to EI (NOx)=0]. For the AER, SLIMCAT, and UNIVAQ models, added NOx
buffers the larger total O3 depletion derived when H2O is the only emitted constituent.
When NOx emission is included, six of the models (CSIRO, GSFC, LLNL, SCTM1,
SLIMCAT, and THINAIR) calculate a larger O3 depletion as EI(NOx) increases from
5 to 15 (S1c-e). The AER model showed little sensitivity to EI(NOx) in this
range. Within the same range, the LARC and UNIVAQ models showed a positive O3
change slope. In general, the model-derived O3 change is in better agreement
among participating models at EI(NOx)=5, with a spread among models from approximately
0% total column O3 depletion (UNIVAQ) down to -0.4% (GSFC and SLIMCAT). At EI(NOx)=15,
the overall spread in model-derived total column O3 change is much larger (+0.4%
to -0.9%).
There is also a large spread in interhemispheric gradients in model-derived
total column O3 change (see Tables 4-11 and 4-12).
For example, when the emission scenario is S1c [EI(NOx)=5 with 500 supersonic
aircraft], the AER, SCTM1, and CSIRO models derived a larger depletion in total
column O3 in the Northern Hemisphere than in the Southern Hemisphere. The UNIVAQ
model had very little change in column O3 in either hemisphere. The LLNL and
THINAIR models derived a similar change in total column O3 in both hemispheres.
However, the ratio of Southern Hemisphere to Northern Hemisphere change in total
column O3 is greater than 1.5 in the GSFC, LARC, and SLIMCAT models. This larger
depletion in the Southern Hemisphere correlates with increased H2O and NOy being
transported to the Southern Hemisphere and the sensitivity of cold aerosol processes
implemented within the GSFC, LARC, and SLIMCAT models.
4.3.3.4. Ambient Surface Area Density Sensitivity
Volcanic activity within the past several decades has greatly modulated ambient
SAD. Two major volcanic eruptions-El Chichón (April 1982) and Mt. Pinatubo (June
1991)-are responsible for most of the observed SAD change. Satellite instruments
(SAM, SAGE I, SAGE II, and SME; e.g., Thomason et al., 1997) have shown that
average SAD in the lower stratosphere between 1979 and 1995 was greater than
that during volcanically clean periods (SA0; WMO, 1992) by a factor of 8 in
the equatorial region and a factor of 2 to 4 at higher latitudes. These enhancements
decay with e-folding time scales on the order of 1 to 2 years (Yue et al., 1991;
Thomason et al., 1997).
For this assessment, we examined the impact of supersonic aircraft at a SAD
of four times the volcanically clean condition (4xSA0). Results from these higher
SAD sensitivity studies are shown in Figure 4-7b. Unlike
the volcanically clean ambient atmosphere (Figure 4-7a),
when the EI(NOx) varies between 0 and 15 (S3a-d), all participating models derived
a positive slope to the Northern Hemispherical change in total column O3. All
models show a strong interference of the H2O only [EI(NOx)=0] total O3 depletion
when NOx emissions are included [EI(NOx)=5]. This response is consistent with
the idea that at higher ambient SAD, NOx catalytic processes are less important,
mainly as a result of conversion of active NOx radicals to nitric acid by heterogeneous
reactions on sulfate aerosols. As supersonic aircraft NOx is enhanced, the additional
NOx interferes with ClOx, BrOx, and HOx odd-oxygen loss processes, reducing
the net total odd-oxygen loss.
It should be noted that the model-derived impact on total column O3 from increasing
ambient SAD by natural causes (approximately 2-3% decreases in Northern Hemisphere
mid-latitude total column O3 change; Solomon et al., 1996) exceeds the model-derived
impact from supersonic aircraft (typically less than 1% Northern Hemisphere
mid-latitude total column O3 change).
4.3.3.5. Sulfate Aerosols and Polar Stratospheric Cloud
Sensitivities
Heterogeneous reactions are important in defining the O3 removal rate in the
stratosphere. These reactions occur on sulfate aerosols and PSC particles, though
sulfate aerosols have the greater influence because of their global distribution.
New sulfate aerosols are produced by aircraft emissions; sulfate particles are
generated by plume processing of part of the emitted SO2/SO3 (Fahey et al.,
1995; Brown et al., 1996; Danilin et al., 1997; Kärcher, 1997; Kärcher and Fahey,
1997). The remaining gas-phase SO2 is then transported on the atmospheric large/global
scale, where it is oxidized to sulfuric acid and condenses onto preexisting
sulfate aerosol particles (Bekki and Pyle, 1992; Pitari et al., 1993; Weisenstein
et al., 1998). Aircraft also emit H2O and NOx and therefore have the potential
to increase the saturation ratios of water vapor and HNO3, so that the size
distribution, mass, and SAD of PSCs may be perturbed (Peter et al., 1991; Pitari
et al., 1993; see Section 3.3.6).
Table 4-13a: H2O changes from HSCT (18 km, ppmv).
|
Scenario |
#HSCTs |
EI(H2O) |
AER |
60°N January
GSFC
|
UNIVAQ |
AER |
60°S July
GSFC |
UNIVAQ |
S1c
S9d |
500
1000
|
1230
1230
|
0.67
1.19
|
0.84
1.49
|
0.57
1.05
|
0.13
0.24
|
0.26
0.47
|
0.14
0.28
|
|
|
Table 4-13b: NOy changes from HSCT (18 km, ppbv).
|
Scenario |
#HSCTs |
EI(NOx) |
AER |
60°N January
GSFC
|
UNIVAQ |
AER |
60°S July
GSFC |
UNIVAQ |
S1c
S1d
S1e
S9d
S9g |
500
500
500
1000
1000
|
5
10
15
5
10
|
0.77
1.57
2.37
1.36
2.77
|
1.24
2.48
3.71
2.14
4.33
|
0.61
1.18
1.74
0.95
2.00
|
0.13
0.28
0.44
0.25
0.54
|
0.15
0.42
0.69
0.15
0.50
|
0.22
0.37
0.51
0.38
0.66
|
|
|
Table 4-13c: PSC1+PSC2 SAD changes from HSCT (12-24 km,
60-90°N, DJF).
|
|
Absolute Change (µm2/cm3) |
% Change |
Scenario |
#HSCTs |
EI(NOx) |
AER
|
GSFC
|
UNIVAQ |
AER |
GSFC |
UNIVAQ |
S1b
S1c
S1d
S1e
S9d
S9g |
500
500
500
500
1000
1000
|
0
5
10
15
5
10
|
0.06
0.10
0.13
0.16
0.19
0.26
|
0.03
0.05
0.06
0.07
0.09
0.12
|
0.03
0.06
0.08
0.09
0.10
0.13
|
22
33
45
57
66
89
|
39
56
70
82
102
137
|
19
37
50
56
62
81
|
|
|
Table 4-13d: PSC1+PSC2 SAD changes from HSCT (12-24 km,
60-90°S, JJA).
|
|
Absolute Change (µm2/cm3) |
% Change |
Scenario |
#HSCTs |
EI(NOx) |
AER
|
GSFC
|
UNIVAQ |
AER |
GSFC |
UNIVAQ |
S1b
S1c
S1d
S1e
S9d
S9g |
500
500
500
500
1000
1000
|
0
5
10
15
5
10
|
0.24
0.34
0.42
0.52
0.64
0.80
|
0.18
0.20
0.22
0.24
0.37
0.42
|
0.17
0.19
0.25
0.27
0.37
0.42
|
4.6
6.5
8.0
10
12
15
|
11
12
13
15
23
26
|
6.7
7.6
10
11
15
17
|
|
|
4.3.3.5.1. Sulfate aerosol sensitivity-SO2 gas-to-particle
conversion in the supersonic aircraft plume
Supersonic aircraft consuming sulfur-containing fuel will have some effect
on sulfate aerosol amounts in the stratosphere (see Section
3.7.3). In this assessment, the increased SAD from sulfur conversion to
particles in the plume had a significant impact on stratospheric ozone. Figure
4-8 shows the percentage change in annual average Northern Hemisphere total
column O3 correlated to the percentage increase in SAD for given SO2 gas-to-particle
conversion assumptions. The percentage SAD increase is derived by averaging
the SAD change relative to the volcanically clean atmosphere (SA0) within the
region between 14-21 km and 33-90°N. The SAD percentage increases for 0, 10,
50, and 100% SO2 gas-to-particle conversion assumptions in the plume are 27,
38, 82, and 111%, respectively. The 0% SAD increase on the abscissa is taken
from supersonic scenario S1c; it represents a perturbation without any consideration
of aircraft sulfur emissions. The AER model was the only model to compute the
0% SO2 gas-to-particle conversion impact on total O3 change. Here, the additional
aircraft-emitted SO2 gas increased the ambient SAD by 27%, altering the total
O3 decrease for the AER model from 0.3 (SA0) to 0.6% (SA7). When particle conversion
was assumed, all participating models derived relatively large decreases in
total O3. In fact, AER and GSFC model results for the Northern Hemisphere showed
O3 depletions of greater than 1% when a 50% SO2 gas-to-particle conversion efficiency
was assumed.
4.3.3.5.2. PSC sensitivity
The change in SAD of PSCs from HSCT aircraft emissions has been calculated
by the AER, GSFC and UNIVAQ 2-D models. The surface area increase is a consequence
of the stratospheric injection of H2O and NOy from aircraft. This process has
the effect of increasing the saturation ratios of both water vapor and nitric
acid, producing an enhancement of PSC particle mass and SAD. Changes in H2O
and NOy from HSCT aircraft at 18 km and 60°N and 60°S are listed in Tables 4-13a
and 4-13b. The average additional SAD of PSC1+PSC2
aerosols in the 12-24 km altitude layer and poleward of 60°N during December-January-February
(DJF) or poleward of 60°S during June-July-August (JJA) are shown in Tables
4-13c and 4-13d. These results should be interpreted
with caution because PSC distributions are highly localized.
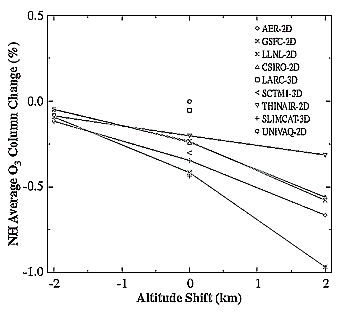
Figure 4-9: Northern Hemisphere total O3 column change as a function of cruise altitude of
the supersonic fleet in 2015 with EI(NOx)=5, SA0 sulfate distribution, and no sulfur aircraft emissions.
|
All three models assume PSC1 to be solid NAT particles, although the treatment
for PSC1 and PSC2 formation varies from model to model. AER and GSFC use a thermodynamic
equilibrium between gas and condensed phase for HNO3 and H2O (Hanson and Mauersberger,
1988), with an imposed size distribution. Supersaturation ratios of 10 and 1.4
are required before PSC1 and PSC2 form in the GSFC model, thus lowering the
temperature threshold for particle nucleation by about 3 and 2 K, respectively.
Denitrification and dehydration are included in the models by calculating HNO3
and H2O loss terms from sedimentation. The UNIVAQ model makes the calculation
by integrating on the NAT/ice particle size distribution; the AER and GSFC models
assume that all condensed HNO3 and H2O form NAT and ice particles of a prescribed
size distribution. No supersaturation is assumed in the AER model. In these
two models, perturbations of sulfate aerosols from aircraft emission of SO2
leave unaffected the PSC. The models do allow H2O and HNO3 changes from aircraft
to affect PSC. The UNIVAQ model allows interaction between sulfate aerosols
and PSC by adopting a microphysical code for both types of particles (Pitari
et al., 1993). As the atmosphere cools, PSC1 particles are first formed by nucleating
on preexisting sulfate aerosols; below the frost point, PSC1 become coated with
ice, thus forming PSC2 particles. With this scheme, a simultaneous presence
of sulfate, PSC1, and PSC2 particles is possible, and size distributions are
calculated for all particle types.
The spread in absolute change of PSC SADs shown in Tables 4-13c
and 4-13d is not surprising because of the different
schemes for PSC formation adopted in the models. In particular, the absence
of supersaturation assumptions in the AER model consistently produces larger
PSC SAD. The spread in relative changes is closely related to different relative
increases of H2O and NOy. About 50-60% (70-90%) of the calculated PSC perturbation
in the Northern Hemisphere (Southern Hemisphere) is caused by HSCT H2O emissions
when EI(NOx)=5. The more important role of H2O emissions in the Antarctic winter
is a consequence of persistent PSC2 particles there; in the Arctic winter, PSC1
particles contribute to almost the entire available PSC SAD.
4.3.3.5.3. Column ozone sensitivity to PSCs
Representation of heterogeneous processes on cold aerosols (PSCs) is not consistent
among the participating models (Sections 4.3.1.3
and 4.3.3.5.). These differences are manifest when increased abundances of NOY and H2O from supersonic aircraft are considered. As mentioned previously,
the transport fields of participating assessment models are significantly different;
therefore, the amount of supersonic H2O and NOy dispersion between hemispheres
and vertically within the same hemisphere is also different. Three models (AER,
GSFC, and UNIVAQ) investigated the impact on total O3 when cold aerosol representation
was not included (S4b). These models removed their representation of SAD, dehydration,
and denitrification that would be associated with cold aerosol processes. The
results were mixed. When cold aerosol processes were included in the model chemistry
formulation, the model-derived percentage change in Northern Hemisphere total
column O3 increased by factors of 1.1 and 1.5 for the GSFC and AER models, respectively
(S1c vs. S4b). However, in the Southern Hemisphere the GSFC model-derived depletion
in total O3 increased by 2.5 when cold chemistry processes were included. There
was minimal change in Southern Hemisphere average O3 depletion in the AER model.
When the EI(NOx) is set to zero (S4a), a similar conclusion is also drawn (compare
to S1b) by the AER and GSFC models. These results are consistent with the dispersion
of supersonic H2O and NOy between the AER and GSFC models. The UNIVAQ model
did show sensitivity to supersonic emissions in the total O3 hemispheric averages;
because the absolute impact was small and centered around zero, however, it
is difficult to draw any significant conclusions.
4.3.3.6. Ambient Chlorine Sensitivity
Total radical chlorine (Cly) abundance in the atmosphere is predicted to decrease
in the future as a result of international agreements controlling production
of anthropogenic organic source gases such as chlorofluorocarbons (CFCs) and
hydrochlorofluorocarbons (HCFCs) (WMO 1995, 1999). Therefore, several sensitivity
studies were performed to investigate the impact of ambient Cly levels on a
given supersonic aircraft perturbation. Here, atmospheric chlorine amounts were
varied by changing halocarbon boundary conditions. Four different ground halocarbon
amounts were considered, which resulted in reactive Cly levels of 1.0, 2.0,
3.0, and 4.0 ppbv in the upper stratosphere. For reference, the 3.0 and 2.0
ppbv Cly levels are representative of predicted 2015 and 2050 atmospheric conditions,
respectively. In all Cly sensitivity studies, only EI(NOx)=5 and a fleet of
500 supersonic aircraft were considered. Sensitivity studies were conducted
under volcanically clean conditions without aircraft sulfur emissions (SA0)
and with sulfur emissions assuming 50% SO2 gas-to-particle conversion in the
plume (SA1). Without aircraft sulfur emissions, there was little discernible
sensitivity to background Cly abundance (S1c, S5a, S6a, and S9a). With sulfur
emissions included, however, total ozone depletion for all three participating
models was smallest when Cly abundance was 1.0 ppbv. As Cly increased in abundance,
the model-derived ozone depletion increased, maximizing and leveling off between
3.0 and 4.0 ppbv Cly (S1f, S5b, S6b, and S9c). Among the participating models,
AER showed the most sensitivity to Cly abundance, followed by GSFC and UNIVAQ.
It should be noted that the Cly=2 ppbv scenario (S9c) had N2O and CH4 boundary
conditions consistent with the year 2050. The other three Cly sensitivity scenarios
(S1f, S5b, and S6b) had N2O and CH4 abundances consistent with the year 2015.
The AER model did investigate Cly sensitivity to N2O and CH4 boundary conditions
by repeating the Cly=2.0 ppbv scenario with N2O and CH4 values representative
of 2015 conditions. The model-derived change in NH total O3 was -0.6% (instead
of -0.7% under 2050 CH4 and N2O conditions).
4.3.3.7. Fleet Design
4.3.3.7.1. Supersonic cruise altitude sensitivity
Figure 4-9 (S1c, S2a, and S2b) shows the effect of
lowering or raising the supersonic cruise altitude by 2 km relative to the baseline
flight cruise altitude band of 17-20 km for an assumed fleet of 500 EI(NOx)=5
aircraft. In most altitude sensitivity studies, ambient SAD was considered to
be volcanically clean, and sulfur emission by HSCT aircraft was not included.
The model-derived change in annual average Northern Hemisphere total column
O3 had a large dependence on emission altitude in participating assessment models.
This cruise altitude impact on O3 highlights the fact that as NOx is emitted
lower in the stratosphere, the residence time of supersonic aircraft emitted
NOx is significantly shorter. In addition, at a lower cruise altitude the chemical
regime is quite different, and past modeling studies have shown that a crossover
point exists where an additional NOx molecule will produce O3 based on the well-known
CH4-smog mechanism (Johnston and Quitevis, 1975). When the cruise altitude is
shifted higher, emissions have a longer stratospheric lifetime and can be transported
more readily to regions dominated by NOx destruction of O3.
Each of these models showed less total column O3 depletion when the cruise
altitude was shifted down by 2 km. Three models also investigated sensitivity
to higher values of EI(NOx) (10 and 15) at the lower cruise altitude (S2c-d).
The model-derived total column O3 change generally increased in the direction
of higher EI(NOx). These results are consistent with the 1995 NASA supersonic
assessment (Stolarski et al., 1995).
Two models (AER and UNIVAQ) did look at sensitivity to cruise altitude with
increased SAD from sulfur emissions as 50% particles (S7c). In this scenario,
an enhanced SAD was not supplied but derived within the 2-D model, coupled with
a sulfate microphysical model. The model-derived annual average Northern Hemisphere
depletion in total column O3 was reduced from -1.0 (S7a) to -0.6% (S7c) in the
AER model and from -0.5 (S7a) to -0.2% (S7c) in the UNIVAQ model when the supersonic
cruise altitude was lowered by 2 km. The opposite impact was derived by the
AER model when the cruise altitude was raised 2 km. Here, the model-derived
annual average Northern Hemisphere depletion in total O3 increased from -1.0
(S7a) to -1.4 % (S7b) in the AER model and from -0.5 to -0.7% in the UNIVAQ
model.
4.3.3.7.2. Supersonic fleet size sensitivity
In this assessment, fleet sizes of 500 and 1,000 supersonic aircraft were considered
at EI(NOx) values of 5 and 10. The increase in fleet size from 500 to 1,000
aircraft included additional routes as well as additional aircraft; as a consequence,
the geographical distribution of emissions changes with proportionally more
flights in the tropics (Baughcum and Henderson, 1998). In addition, by increasing
the fleet size, the amount of H2O emitted is also increased proportionally.
For most of the cases considered, doubling the fleet size had a nearly linear
effect on the Northern Hemisphere column O3 reduction. The exception is the
UNIVAQ model, which showed almost no change with increasing fleet size. For
the 2050 atmosphere with aircraft sulfur emission as 50% particles, increasing
the fleet size from 500 to 1,000 aircraft has a less than linear effect, especially
with the AER model.
Table 4-14a: Mid-latitude lower stratospheric mass density(MD,
ng/cm3) and SAD (µm2/cm3) with/without subsonic aircraft in 2015 [annual
average, EI(SO2)=0.4 g/kg, 10-14 km, 30-60°N).
|
|
No Aircraft |
50% Particles |
100% Particles |
|
Model |
MD |
SAD |
|
MD |
SAD |
|
MD |
SAD |
AER
UNIVAQ |
48.3
59.0 |
1.0
0.7 |
|
55.0
63.5 |
1.6
2.4 |
|
59.2
65.7 |
2.1
3.2 |
|
|
|
Table 4-14b: Global and mid-latitude ozone column changes
from
subsonic aircraft in 2015 (annual average, %).
|
|
No Sulfate Emission |
50% Particles |
100% Particles |
|
Model |
Global |
45°N |
|
Global |
45°N |
|
Global |
45°N |
AER
UNIVAQ |
0.79
0.30 |
1.29
0.72 |
|
0.57
0.14 |
0.94
0.29 |
|
0.51
0.07 |
0.86
0.15 |
|
|
|
|