4.4. Uncertainties in Model Results
It is not clear how we can properly represent the true uncertainty associated
with atmospheric effects; such an assessment represents an unattainable goal
for the present. The model results presented in this chapter have been calculated
using state-of-the-art atmospheric models from many groups. As a result of their
different treatment of physical, dynamic, and chemical processes, there is a
considerable range in their computed impacts. We have attempted to bracket the
results by using a combination of the range of different results between models
for the same scenarios and results from sensitivity tests of parameters judged
to be important.
Many factors contribute to the uncertainty of model calculations for present
and future descriptions of the atmosphere. These factors include deficiencies
in the representation of transport processes (e.g., stratosphere/troposphere
exchange and tropical and polar region barriers); model resolution; the dimensionality
of the models (i.e., 2-D or 3-D); deficiencies in chemical and physiochemical
processes; and the state of the future atmosphere. Many of these issues cannot
be considered in isolation. For example, the spatial resolution of a model may
determine how well it is able to capture the meteorological details required
for an accurate simulation of the dispersion of plumes and the formation of
PSCs. Furthermore, as noted earlier, the models we used for our calculations
can be considered to be of two types: Those that have been formulated for a
detailed simulation of the troposphere, and those designed to simulate primarily
stratospheric processes. This use of two model types to attack a problem whose
resolution requires the ability to model the troposphere and stratosphere adds
further uncertainty to the results obtained.
It should also be noted that sensitivity studies from the 3-D tropospheric
subsonic scenarios are often based on runs performed with a single model, thus
should be viewed with caution. In other cases, such as that of sensitivity to
NMHC chemistry, the different models had different sensitivities.
As discussed in Chapter 2, time constants for transport in the stratosphere
can be very long, and models must be run for many years to calculate the background
state and the perturbed atmosphere. In addition, the parameter space that must
be probed for unbuilt HSCTs is much more extensive than for the characteristics
of the subsonic fleet. The use of 2-D models allowed for a more detailed analysis
of the parameter space associated with supersonic aircraft. However, the results
from these runs are also limited by the 2-D nature of the models.
Some of the uncertainty issues noted above have been discussed from a somewhat
different perspective in Section 2.3. In addition, several
international assessment reports (Stolarski et al., 1995; WMO, 1995; Fabian
and Kärcher,1997; Friedl, 1997; Brasseur et al., 1998) have addressed some of
these uncertainties. In the following paragraphs, we describe some features
related to model uncertainty that we have identified in this study.
4.4.1. Uncertainties in Calculated Effects of Subsonic
Aircraft Emissions
4.4.1.1. Transport and the Fuel Study
One means of assessing model uncertainties from transport is by comparing concentrations
of inert or passive tracers with specified input and removal rates. For such
species, it may be assumed that differences in distribution are solely a result
of transport and its representation because chemistry plays no role. The results
of such an experiment are described in Section 3.3.4.1
and Danilin et al. (1998). Because the emissions in this experiment are based
on fuel use by subsonic aircraft, the experiment tests the transport characteristics
of models in the UT and LS. Mixing ratios of fuel tracer are roughly 10-100
ng/g between 8-16 km north of 30°N. Southern Hemisphere stratospheric concentrations
are about a factor of 4 less than in the Northern Hemisphere. Although the general
pattern of the test tracer is qualitatively similar among various models, the
maximum tracer concentrations deviate by a factor of 10 among the models. Some
of this difference may be an artifact of low vertical resolution, but the results
clearly point to uncertainties that need to be resolved.
One of the major problems with model tranport is that stratospheric-tropospheric
exchange (STE) is poorly represented in current models of any dimension. Current
theory has air entering the stratosphere through the tropics and returning at
mid-latitudes. However, recent measurements of chemical tracers such as H2O
and CO, diagnostic of tropospheric air, suggest that the lowest few kilometers
of the lowermost stratosphere can be impacted by air being convected from the
lower troposphere (Dessler et al., 1995; Lelieveld et al., 1997). These areas
require more evaluation.
One of the problems that has not been addressed in these studies is sub-grid-scale
parameterizations. This issue includes dispersion of material in the plume to
the regional scale, which is typical of the grid resolution of most models.
In addition, exchange of air between the troposphere and the stratosphere takes
place on a variety of scales, and only the larger scales are modeled with any
reliability.
Thus, it is very difficult to assess inherent uncertainties in model results
that are products of limitations in modeling transport. Nevertheless, as explained
in Section 4.5, we attempt such an assessment by comparing
the different models in a semi-quantitative manner.
4.4.1.2. Chemistry
The chemistry of VOCs (NMHC, acetone) and the transport of peroxides to the
upper troposphere have recently been found to play important roles in the HOx
radical balance (Singh et al., 1995; Folkins et al., 1997; Jaeglé et al., 1997;
McKeen et al., 1997; Prather and Jacob, 1997; Wennberg et al., 1998). An additional
source of HOx in the UT could significantly influence O3 production efficiencies.
This uncertainty would propagate through the 3-D tropospheric models because
there are large differences in chemistry modules. To some degree, this effect
has been explored and the results tabulated in Table
4-6. Further model studies clearly are needed.
NOx plays a key role in the O3 generation process in the UT and LS, and there
are significant differences in predicted NOx levels among the models. However,
because of the limited number of observations and the large temporal and spatial
variations of these observations, it is difficult to validate modeled upper
tropospheric and lower stratospheric concentrations (see Chapter 2).
Although the process studies referred to in Chapter 2 point to a strong dependence
of O3 production on NOx levels, the standard simulations and sensitivity studies
presented in Chapter 4 show a limited impact of nonlinear chemistry on O3 perturbations.
For example, all of the 3-D CTM standard simulations for 1992, 2015, and 2050
calculated a nearly linear increase in O3 perturbation as a result of increases
in aircraft NOx emissions despite increases in background NOx levels. As noted
above, there are large differences among models in their calculation of O3 transport
and chemistry between the UT and LS, and it is in this region where O3 has a
significant contribution to the infrared heating budget (Wang and Sze, 1980;
Lacis et al., 1990; Fortuin et al., 1995).
Given the limitations of the tropospheric models to estimate O3 perturbations
in the tropopause region-in particular perturbations in the LS-a comparison
of fractions of O3 change that occur in the troposphere is made. Considering
different characteristics of each model, meteorological and numerical, the estimated
tropopause height may differ considerably among them. In addition, the "real"
tropopause height may vary locally by at least 2 km, depending on the season
and the local meteorology. Thus, we have applied the following crude estimate
of tropopause height to all models: 8 km poleward of 65°, 10 km between 65°
and 50°, 12 km between 50° and 35°, 14 km between 35° and 25°, and 16 km in
the tropics. These figures are consistent with average tropopause heights used
by the Total Ozone Mapping Spectrometer (Fishman et al., 1990).
The fraction of O3 change from 1992 aircraft emissions that occurs in the troposphere
varies between 0.65 and 0.75, with an uncertainty of about 15%. The fraction
calculated for the stratospheric 2-D AER model was 0.81. The ECHAm3/CHEM model
predicts the lowest tropospheric fraction (0.65). As noted above, the assumption
is that this fraction of 0.65 represents minimum O3 generation in the troposphere
from subsonic emissions by the current (1992) fleet.
However, the model simulations just discussed did not include the generation
of sulfate by aircraft, and consideration of this factor may alter the picture
somewhat. Because no 3-D tropospheric models were available to investigate sensitivity
to sulfate emissions, an attempt to assess their significance was made using
the AER and UNIVAQ 2-D microphysical models. Although these models are limited
in their applicability to the UT, they do yield results that suggest further
work is required to evaluate the significance of calculated O3 generation by
subsonics.
Aircraft sulfur experiments have been carried out for 2015 using an EI(SO2)=0.4
for a fleet flying nominally at 10 km. It was further assumed that the SO2 emitted
was converted in the plume to 10 nm (radius) particles with 50 and 100% efficiency.
Table 4-14a summarizes the model predictions of
aerosol increases at middle northern latitudes for the 10-14 km height range.
The importance of transport is clear: Although new particles are formed at
about 11-km altitude, the models yield significant changes of SAD up to about
14 km, thus creating a potentially important link between subsonic emissions
and stratospheric O3. This conclusion is consistent with the results of the
1992 tracer experiment (see Section 3.3.4), in which
2-D and 3-D models calculate the largest fuel tracer mixing ratios in an altitude
layer from about 10 to 14 km.
Table 4-14b gives concomitant O3 column changes.
The AER and UNIVAQ models show that the O3 column increase from atmospheric
NOx aircraft perturbation tends to be mitigated when sulfate SAD is changed
by the aircraft. This result is mainly a consequence of more heterogeneous reactions
on sulfate particles, leading to the release of ClO, BrO, and HO2 in this layer.
However, the reduction in magnitude of O3 generation is much stronger in the
UNIVAQ model as compared to the AER model. Clearly, this issue requires more
study.
4.4.2. Uncertainties in Calculated Effects of Supersonic
Aircraft Emissions
4.4.2.1. Transport
Clearly, an important uncertainty in the evaluation of the effects of aircraft
is the representation of transport in the 2-D and 3-D models used to simulate
the effect of supersonic aircraft emissions. The research community recognizes
this general limitation, and an ongoing exercise (Models and Measurements II,
Park et al., 1999) should help to quantify some of the transport differences
among the models. Models and Measurements II is an activity supported by NASA
to test models through detailed comparisons with measurements. It is a follow-on
of the Models and Measurements workshop completed several years ago (Prather
and Remsberg, 1993). The results of Models and Measurements II should be available
by the end of 1999.
New analysis techniques of models relying on "age of air" (Hall and Plumb,
1994), sulfur hexafluoride (SF6) (Elkins et al., 1996), and CO2 (Boering et
al., 1996) measurements allow more detailed comparisons to test the transport
of models. Most models tend to underestimate the observed "age of air;" therefore,
the derived delta NOy and H2O for these models is probably a lower limit. As
a result, the models will tend to underestimate O3 depletion from supersonic
aircraft.
Another measure of transport uncertainty is illustrated by significant differences
in mixing ratios calculated for NOy accumulation in the stratosphere from supersonic
aircraft emissions (Figure 4-6a). This uncertainty
reflects differences in lower stratospheric residence times for the different
models.
The atmosphere undergoes large variations from one year to the next. These
interannual variations will drive a different transport of constituents, which
will lead to significant differences in the impact of supersonic emissions on
O3. Jackman et al. (1991) simulated very different aircraft-induced O3 changes
using separate dynamical fields; a slow (rapid) circulation simulation caused
larger (smaller) O3 decreases. The SLIMCAT model has been used with a yearly
varying transport recently and has computed total O3 decreases that vary significantly
in the same months of different years.
4.4.2.2. Model Resolution/Dimensionality
As noted above, the formidable computational resources required to conduct
long-term multiyear 3-D stratospheric simulations for assessment has made it
necessary to use 2-D models. Although the 2-D models have very sophisticated
formulations for gas phase chemical processes, their meridianal resolution is
typically 5° (550 km) or coarser in latitude and 1 km or more in altitude throughout
the stratosphere. Intrinsically, this resolution limits their ability to treat
LS transport processes. In addition, because atmospheric species are treated
in a zonally averaged manner, effects such as PSC formation and chemical processing
must be parameterized more crudely than in 3-D models.
Issues of spatial resolution and dimensionality of models are very pertinent
to the treatment of PSCs and the processing of polar air. 2-D models do not
adequately parameterize the zonal variation of temperature, thus the formation
of PSCs. Even 3-D models do not capture the different horizontal temperature
scales within the Arctic stratosphere. For example, even though synoptic conditions
would suggest otherwise, low temperatures and PSCs may be induced by wave flow
over mountains (Carslaw et al., 1998); this mesoscale phenomenon is not directly
addressed by most models to date. This uncertainty in assessing impacts from
aircraft could be an even more important issue in trying to assess future impacts
when the stratosphere may be cooler because of enhanced CO2 mixing ratios (Shindell
et al., 1998).
Yet another, quite different, resolution problem that may limit our ability
to model chemical effects is that of filamentation processes, whereby winter
polar air consists of long but narrow (< 5 km) filaments of gas in which species
densities are different. O3 depletion studies performed at very high horizontal
resolution for the winter Arctic (Edouard et al., 1996) have underlined the
uncertainty in calculating chemical loss of O3 from differing resolutions.
4.4.2.3. Stratospheric Chemistry
Current models do quite well in reproducing the general behavior of O3 in the
stratosphere. Nevertheless, as is evident from the discussion in Chapter 2 and
above, problems remain. For example, there appears to be a discrepancy of about
30% between calculated and measured O3 levels near the stratopause (Osterman
et al., 1997). In addition, related problems emerge with a careful comparison
with observations, such as the ratio of active to reservoir species for Cly
(Michelsen et al., 1996). These problems may be alleviated by the inclusion
of new HCl and hydrogen bromide (HBr) formation rates (see Chapter 2).
Table 4-15a: Carbon soot mass mixing ratio from future
aircraft [(50°N, annual average, pptm, EI(soot)=0.04 g/kg); approximate
conversion factors to ng/m3 are 0.3 at 12 km and 0.1 at 20 km].
|
|
2015
Subsonic
|
2015
Subsonic + HSCT
|
2050
Subsonic
|
|
Model |
12 km |
20 km |
|
12 km |
20 km |
|
12 km |
20 km |
AER
ECHAm3/CHEM
Tm3/KNMI
UiO
UNIVAQ-2D
UNIVAQ-3D |
2.0
0.9
1.7
2.6
3.0
2.9 |
0.6
0.4
0.4
0.7
0.2
0.5 |
|
4.8
5.1
4.1
3.7 |
10.8
9.1
9.5
9.2 |
|
2.8
1.4
3.8
4.6
4.5 |
0.9
0.6
1.0
0.3
0.8 |
|
|
|
Table 4-15b: Carbon soot column from future aircraft (50°N,
annual average, ng/cm2, EI(soot)=0.04 g/kg).
|
Model
|
2015
Subsonic
|
2015
Subsonic + HSCT
|
2050
Subsonic
|
AER
Tm3/KNMI
UiO
UNIVAQ-2D
UNIVAQ-3D |
0.58
0.44
0.68
0.70
0.69
|
2.18
1.53
1.70
1.52
|
0.84
1.00
1.06
1.04
|
|
|
The models generally assume the same set of reaction rate constants and photodissociation
cross-sections based on the DeMore et al. (1997) recommendations. Comparisons
of results for a subset of models (AER, CSIRO, GSFC, LARC, LLNL, SLIMCAT) in
a benchmark at photostationary steady-state (Stolarski et al., 1995) indicate
that the differences were less than 1% for CSIRO and LLNL; a few percent for
AER, LARC, and SLIMCAT; and a few percent up to 10-15% for GSFC. Similar results
are not available for the other models, and it is not possible to conclude that
the chemical solvers in all models are equivalent throughout the atmosphere;
however, the choice of chemical solver does not appear to be a major uncertainty
in our ability to perform assessment calculations.
Uncertainty in photochemical rate constants has been propagated through a 2-D
assessment model using a Monte Carlo approach for an EI(NOx)=15 HSCT fleet with
background aerosols (SA0) and no sulfur emissions (Stolarski et al., 1995).
The effect of uncertainties in gas-phase reaction rate coefficients on perturbutions
of O3 are estimated to yield an uncertainty on the order of 1% (1-sigma) in
calculated Northern Hemisphere O3 column perturbations. However, these experiments
did not include uncertainties in photolytic cross-sections and heterogeneous
processes, which could enhance the uncertainty significantly. Recently, box
model sensitivity-uncertainty calculations for O3 depletion from supersonic
aircraft emissions, again with EI(NOx)=15 and background aerosols, were performed
at the most perturbed locale using localized outputs from a 2-D model (Dubey
et al., 1997). Guided by these sensitivities, 2-D model integrations were completed
with nine targeted input parameters altered to 1/3 of their 1-sigma uncertainties
to put error bounds on the predicted O3 change. Results indicated local O3 loss
of 1.5 ± 3% in regions of large NOx injections. We note, however, that these
sensitivity studies were all carried out with relatively high EI(NOx) values
[three times the EI(NOx) taken as standard for the current assessment], which
is likely to further modify the intrinsic sensitivities of the models to perturbations.
4.4.2.4. Polar Stratospheric Cloud, High Cirrus Cloud,
and Aerosol Processing
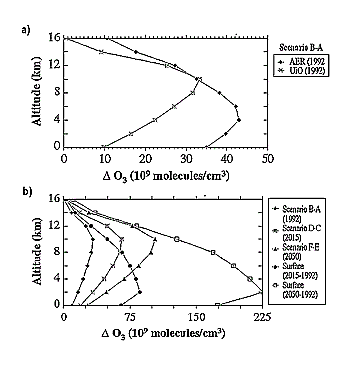
Figure 4-10: Annual average zonal mean change of ozone in the 30-60°N latitude band for
various perturbations from UiO-3D and AER-2D models. Predictions are shown for (a) both models in 1992;
and (b) UiO-3D model in 1992, 2015, and 2050.
|
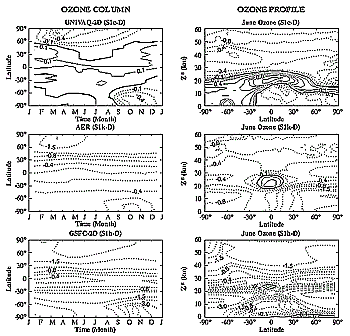
Figure 4-11: Ozone column (left panels) and ozone profile (right panels) percentage
difference as a function of latitude and month for three models and three supersonic aircraft
scenarios with respect to scenario D: UNIVAQ-2D model for S1c; AER-2D model for S1k; and GSFC-2D
model for S1h. This figure mainly illustrates model differences rather than scenario differences.
|
Uncertainty in our knowledge of the present atmosphere affects our ability
to calculate formation of and the chemical processing associated with PSCs.
This uncertainty is particularly acute in the Arctic winter because early springtime
temperatures are higher in the Arctic than in the Antarctic, and are quite close
to the threshold temperature for the formation of PSCs. Thus, a temperature
error of a few degrees can make a substantial difference in calculating the
extent of processing. Objectively analyzed temperatures-those based on measurements
and often processed for use in weather forecast models-are frequently used in
3-D CTMs to estimate chemical processing. However, it appears that UKMO and
ECMWF objectively analyzed temperatures may be systematically too high by 1
to 2 K in winter (Knudsen, 1996; Pullen and Jones, 1997); this uncertainty will
impact our understanding of the microphysics associated with heterogeneous chemistry.
The question of processing of reservoir species such as HNO3 and HCl on PSCs
remains uncertain, and hysteresis effects may be important. That is, the formation
of PSCs by condensation and removal by evaporation may not be describable in
terms of reversible processes, and the temperature history of the air parcel
may be important. Currently, most models avoid this complication. Conversion
of reservoir species may occur as a result of dissolution of HCl in sulfate,
forming a ternary solution, or may occur on frozen surfaces of water or NAT
ice surfaces. The sensitivity of O3 depletion to the details of these processes
appears to be less important for Antarctic conditions; many models yield relatively
complete conversion of reservoir species induced by cold vortex temperatures.
However, as noted above, this effect could be more important for the relatively
warmer Arctic vortex. This uncertainty associated with chemistry, combined with
the dynamic uncertainty, suggests that modeling of the propagation of PSC processing
effects onto global scales is very uncertain.
Solomon et al. (1997) pointed out that the presence of high cirrus clouds near
the tropical tropopause could decrease O3 in the stratosphere by activating
chlorine constituents. Emissions of H2O and NOx from supersonic and subsonic
aircraft have the potential to increase the occurrence of high cirrus clouds
(see Chapter 2). This effect is not evaluated by the model simulations performed
in this chapter.
4.4.2.5. Underlying Subsonic Fleet
In all scenarios for 2050 except two (S11a and S12a), the subsonic fleet was
as defined for the 2015 scenarios. For scenarios S11a and S12a, a more realistic
representation of the subsonic fleet in 2050 was used. For example, scenario
S11a was compared with the IS92a predicted subsonic fleet scenario F (scenario
Fa1 in Chapter 9), and scenario S12a was compared with the high-demand subsonic
fleet scenario G (scenario Fe1 in Chapter 9). As for all other supersonic scenarios,
S11a and S12a contain subsonic aircraft emissions as well as HSCT aircraft emissions;
the combination accounts for the same passenger demand as in subsonic-only scenarios
F and G for 2050. Scenarios S9d, S11a, and S12a have similar supersonic components,
but the subsonic components in the perturbed and the base runs (D9, F, and G,
respectively) are very different. There are only minor differences in predicted
total column O3 change for a particular model in either hemisphere related to
assumptions about the underlying subsonic fleet size (Table
4-12). Therefore, the size of the underlying subsonic fleet is computed
to have only a minor impact on predicted O3 changes from the projected supersonic
fleet.
4.4.2.6. Future Atmosphere
An additional uncertainty associated with the calculation of future aircraft
impact is related to the increasing trend of greenhouse gases in the atmosphere
and the impact on stratospheric temperatures and transport. For example, a CO2
mixing ratio of about 500 ppmv is the recommendation of WMO (1992) for CO2 in
the year 2050-about 1.5 times the 1980 value. The change in radiative forcing
as a result of greenhouse gas increases will affect stratospheric temperature
and circulation (see Chapter 12 of WMO, 1999, and references therein); this
effect has not been taken into consideration here. The likely cooler future
stratosphere would delay the recovery of polar O3 in Arctic and Antarctic regions,
which could affect the future impact of aircraft.
One study of the effect of increasing CO2 amounts (Pitari and Visconti, 1994)
showed that stratospheric cooling mitigated the increase in NOx. However, this
study did not take into consideration the increase in H2O associated with aircraft;
therefore, more complete studies need to be done with a wide range of models.
Another major uncertainty regarding knowledge of the future stratosphere is
that associated with source gases, in particular H2O. This uncertainty is underscored
by our lack of understanding of current H2O trends. Global trends in stratospheric
humidity over the period 1992 to 1996 inclusive have been presented recently
by Evans et al. (1998) using Upper Atmosphere Research Satellite (UARS) measurements
of H2O and CH4 from the Halogen Occultation Experiment (HALOE) instrument. The
authors find that the combined budget of 2CH4 + H2O is approximately constant
with altitude and is increasing with a global mean value of 61 ppbv yr-1. A
more local view of water vapor increase in the lower stratosphere was presented
by Oltmans and Hofmann (1995) over the period 1981 to 1994. They found a maximum
rate of increase in the 18-20 km layer of 32 ppbv yr-1. The rate of increase
of H2O observed in the stratosphere is greater than the increase in tropospheric
CH4. One suggestion is that the increase could be accounted for by a change
in tropopause temperature of a few tenths of a degree, and the most recent changes
might be a result of changes in the dynamics of the tropics induced by heating
of Mt. Pinatubo aerosols (Schauffer and Daniel, 1994). This uncertainty highlights
the delicate balance between chemistry and climate.
4.4.3. Soot Emissions by Subsonic and Supersonic Aircraft
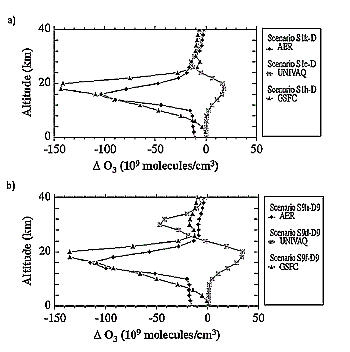
Figure 4-12: Annual average zonal mean change of ozone in the 30-60°N
latitude band for supersonic aircraft perturbations from AER-2D, GSFC-2D, and UNIVAQ-2D
models in (a) 2015 and (b) 2050. Model scenarios are those passed through to Chapters
5 and 6.
|
The chemistry of soot has been discussed in Chapter 2; the conclusion is that
it does not react with O3 in a catalytic manner. That is, O3 can be destroyed
by soot, but the soot is consumed in the process (see Section
2.1.3.1). However, soot particles may act as condensation nuclei for sulfate
or other species, modifying cirrus clouds in the UT or LS, and locally they
may be important in the destruction of O3 (Bekki, 1997; Lary et al., 1997).
Thus, we present some estimates of soot densities and SADs for future scenarios
to complement those estimated for the current (1992) subsonic fleet already
given in Chapter 3. There are large uncertainties with regard to the sources
and distribution of soot particles. Emissions from aircraft are particularly
uncertain. Few global modeling studies are available for these aerosols (see
Chapter 3 for details). Emissions of soot by aircraft [EI(soot)=0.03-0.4 g/kg]
(Friedl, 1997) have been studied by Bekki (1997), who used the Cambridge 2-D
aerosol/soot model for past and present air traffic, and more recently by Rahmes
et al. (1998) for current and future aircraft fleets.
For the results presented herein, the aircraft-generated soot distribution
was approximated by scaling the distribution of passive tracer calculated according
to aircraft fuel burn (see also Chapter 3). A number of models have calculated
this distribution for 2015 and 2050 (1992 distribution is shown in Table
3-4); all show the largest mass mixing ratio at about 12-km altitude and
poleward of 40°N. The results for subsonic aircraft in the years 2015 and 2050
(IS92 Scenario 1) are shown in Table 4-15. For
2015, some results are also included for subsonic and HSCT aircraft together.
These numbers represent an upper limit because sedimentation is neglected. Soot
particles could also be lost by coagulation with larger sulfate aerosols and
by condensation of H2SO4-H2O on the particle surface.
Taking 2.0 pptm as a reference mixing ratio for subsonic aircraft soot in 2015
at 12 km and 50°N, an approximate SAD of 0.03 µm2 cm-3 is obtained when assuming
(as a first approximation) spherical particles of 2 g cm-3 density (Pueschel
et al., 1997) and a bimodal size distribution peaked at 20 and 100 nm radii
with equal contribution in mass. If the particles are not assumed to be spherical
but can be represented by fractal geometry, their SAD could lead to an enhancement
by a factor of about 4 (Jennings et al., 1999) over the spherical case. This
seems more appropriate than 30, as suggested by Blake and Kato (1995). DeMore
et al. (1997) recommend reaction efficiencies for O3 decomposition between 0.003
and 1 x 10-5, with one O2 molecule produced for each O3 reacting. As noted above,
this O3 decomposition is not catalytic because reaction sites on soot are rendered
inactive when they are used or when the particle becomes coated with H2SO4.
|