3.6. Radiative Properties of Aerosols, Contrails, and Cirrus Clouds
Aircraft emissions have an impact on the Earth's radiation budget and climate
through direct and indirect changes in aerosols and cloudiness. Recent climate
assessments have stressed the importance of natural and anthropogenic changes
in aerosols on direct radiative forcing (Charlson et al., 1990; Schwartz, 1996).
Aerosols and contrails have direct effects (scattering and absorbing solar and
longwave radiation) and indirect effects (modifying the formation of cloud particles
and radiative properties of clouds). Several studies have addressed the direct
impact of contrails (e.g., Detwiler and Pratt, 1984; Grassl, 1990; Liou et al.,
1990; Sassen, 1997). The indirect effect of contrails has not yet been investigated
in detail. The direct radiative impacts of aircraft soot emissions (Pueschel
et al., 1992, 1997) and sulfate aerosol have been evaluated as being small (Friedl,
1997; Brasseur et al., 1998). The indirect radiative effect of aircraft-induced
aerosols on clouds is essentially unknown. In fact, the indirect radiative effect
of non-aviation aerosol has been studied for liquid water clouds (IPCC, 1996),
but the indirect effect of changing cirrus is not yet known either. Here, the
discussion focuses on the impact of aircraft-generated aerosol and that of contrails
and changed cirrus clouds.
Table 3-7: Sensitivity of daily mean of instantaneous net
forcing at top of the atmosphere by contrails to a range of values for various
parametersa for 100% contrail cover. The first two rows contain results
from models FL, M, and N; the others are from model N.
|
Case |
|
Optical Depth
t at 0.55 µm |
Net Forcing
(Wm-2) |
|
|
|
|
Reference (models FL, M, and N) |
|
0.52
|
37.1-37.2
|
Different aspherical particles (models FL, M, and N) |
|
0.4
|
22-36
|
|
|
|
|
Parameters |
Range
|
|
|
|
|
|
|
Solar zenith angle |
60°-21°
|
0.52
|
37-49
|
Ice water content (IWC) |
7.2-42 mg m-3
|
0.2-1.0
|
19-51
|
Particle diameter |
10-40 µm
|
0.85-0.21
|
41-20
|
Surface temperature |
289-299 K
|
0.52
|
35-39
|
Cloud cover/optical depth of underlying clouds |
0-1 / 0-23
|
0.52
|
37-40
|
Surface albedo |
0.05-0.3
|
0.52
|
31-40
|
Relative humidity |
reference - 80%
|
0.52
|
37-31
|
Contrail vertical depth (for fixed ice water path) |
200 m - 1 km
|
0.52
|
37.1-36.7
|
Lower contrail top (for fixed IWC) |
11-10 km
|
0.52
|
37-31
|
Lower contrail top (for temperature-dependent IWC) |
11-10 km
|
0.52-1.32
|
37-45
|
|
|
a) Details in Meerkötter et al. (1999). Reference case for
mid-latitude summer (McClatchey et al., 1972) as in Table
3-6; spherical particles; solar zenith angle of 60°; diurnal sunshine
fraction of 50%; IWC of 21 mg m-3; volume mean particlediameter of 32 µm;
surface temperature of 294 K; no low-level clouds; relative humidity of
reference profile (McClatcheyet al., 1972) varying from 77% at the surface
to 11% at 12-km altitude; contrail depth 200 m; and contrail top at 11-km
altitude. |
|
Radiative forcing is defined as the net radiative flux change at some level
in the atmosphere calculated in response to a perturbation, such as a change
in cloud cover. The definition of radiative forcing used here is the "instantaneous"
or "static" flux change at the top of the atmosphere (TOA) (IPCC, 1996) (see
Chapter 6). A positive net flux change represents an
energy gain, hence a net heating of the Earth system.
3.6.1. Direct Radiative Impact of Aerosols
The direct radiative impact of aircraft-induced aerosol is much smaller than
that of volcanic stratospheric or regional tropospheric aerosol and smaller
than other aircraft-induced radiative forcing values (see Chapter
6). Radiative forcing by aerosol depends on many parameters (Haywood and
Ramaswamy, 1998). For aerosol in the size range 0.1 to 1 µm, the increase in
solar reflectance (albedo effect) exceeds the trapping of terrestrial radiation
(greenhouse effect), causing a surface cooling (Lacis et al., 1992, Minnis et
al., 1993; Minnis et al., 1998c). Aircraft-induced aerosols could have an importance
similar to that of natural stratospheric aerosol variations if their optical
depth in the solar range were of comparable magnitude to that of natural stratospheric
aerosol. The optical depth of aerosol is proportional to its column load. The
maximum zonally averaged column load from soot emissions and aircraft-induced
sulfuric acid is estimated (see Section 3.3) to be less
than 1 and 6 ng cm-2, respectively. Small sulfuric acid particles are nonabsorbent
but scatter strongly in the shortwave spectrum, with mass scattering efficiencies
of 7 (4-10) m2 g-1 in the solar range depending on relative humidity (Boucher
and Anderson, 1995; Lacis and Mishchenko, 1995). Soot is a strong absorber of
solar energy, with mass extinction coefficients of about 10 m2 g-1 (Pueschel
et al., 1992; Penner, 1995; Petzold and Schröder, 1998). As a consequence of
the product of given column load and extinction efficiency, the maximum change
in zonal-mean optical depth from aircraft soot and sulfate aerosol is less than
4 x 10-3. For comparison, the solar optical depth of stratospheric aerosol varies
typically between 0.005 and 0.15, depending on volcanic aerosol loading (Sato
et al., 1993). Regionally, within the main flight corridors, a particle concentration
change of approximately 30 cm-3 at 0.1-µm mean diameter over a vertical layer
of 2 km cannot be ruled out (Schumann et al., 1996; Friedl, 1997; Schlager et
al., 1997), implying a mass load on the order of 10 ng cm-2 and a solar optical
depth of about 0.001. This regional change is small compared with other regional
variations. Tropospheric aerosol layers with optical depth of 0.1 to 0.5 occur
frequently off the coasts of North America and Europe (Russell et al., 1997).
3.6.2. Radiative Properties of Cirrus Clouds
Contrails are ice clouds with radiative effects similar to thin cirrus cloud
layers (Liou, 1986; Raschke et al., 1998). At the TOA, thin layers of cirrus
clouds or contrails tend to enhance radiative forcing and hence the greenhouse
effect because they cause only a small reduction of the downward solar flux
but have relatively larger impact on the upward terrestrial radiative flux.
An increase in cloud cover by thin cirrus clouds may therefore cause an increase
in the net energy gain of the planet (Stephens and Webster, 1981; Fu and Liou,
1993). Contrails and cirrus clouds also have an impact on the radiative energy
budget at the Earth's surface. Although radiative forcing at the TOA is most
important for long-term and global climate changes, forcing at the surface may
have short-term regional consequences.
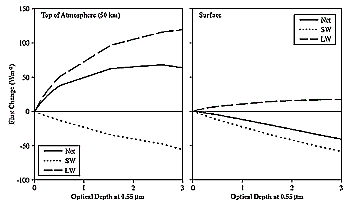
Figure 3-20: Shortwave (SW), longwave (LW), and net instantaneous
radiative flux change from contrails with 100% cover under mid-latitude summer
conditions averaged over a day as a function of optical depth (0.55 µm): Near
the top of the atmosphere (50 km) and surface (0 km) (from Meerkötter et al.,
1999).
|
Longwave (LW) radiative forcing by cirrus or contrails is greatest when clear-sky
radiative flux to space is large (i.e., larger over warm than over cool surfaces,
larger in a dry than in a humid atmosphere) and cloud emissivity is large (Ebert
and Curry, 1992; Fu and Liou, 1993). For thin clouds, emissivity increases with
ice water path, which is the product of the ice water content (IWC) of the cloud
and its geometrical depth. The emissivity of ice particles in a cirrus layer
is much larger, in particular at 8 to 12 µm (King et al., 1992; Minnis et al.,
1998c), than that of the same amount of water in gaseous form. Hence, absorption
and emission from an atmospheric layer increase when ice particles form at the
expense of ambient water vapor (Detwiler, 1983; Meerkötter et al.,1999).
Shortwave (SW) radiative forcing of cirrus clouds is determined mainly by solar
zenith angle, surface albedo, and cloud optical depth (which increases with
ice water path) (Ebert and Curry, 1992; Platt, 1997). For fixed ice water path,
clouds containing smaller particles have larger optical depth and exhibit larger
solar albedo (Twomey, 1977; Betancor-Gothe and Grassl, 1993). Aspherical particles
cause a larger albedo than spherical ones (Kinne and Liou, 1989; Gayet et al.,
1998). SW forcing is negative when the cloud causes an increase of system albedo.
In general, SW forcing has a greater magnitude over dark surfaces than over
bright surfaces.
The net radiative forcing of clouds is the sum of SW and LW flux changes and
may be positive or negative. Thin cirrus clouds cause a small but positive radiative
forcing at the TOA; thick cirrus clouds may cause cooling (Stephens and Webster,
1981; Fu and Liou, 1993). In the global mean, an increase in cirrus cloud cover
warms the Earth's surface (Hansen et al., 1997). Maximum heating is reached
at intermediate ice water path, corresponding to an optical depth of about 2
to 3, and the effect shifts to small cooling for optical depths greater than
about 10 to 20 (Platt, 1981; Jensen et al., 1994a). Net forcing also varies
with particle size but less than its two spectral components. For thin cirrus,
smaller particles (but > 3-mm radius) tend to cause stronger heating by increasing
cloud albedo less strongly than emissivity (Fu and Liou, 1993).
3.6.3. Radiative Properties of Contrail Clouds
Contrails are radiatively important only if formed in ice-supersaturated air,
where they may persist and spread to several-kilometer lateral widths and a
few hundred meters vertical depth (Detwiler and Pratt, 1984; Jäger et al., 1998)
(see also Section 3.4.4). In contrast, shorter lived
contrails have much smaller spatial and temporal scales, hence contribute much
less to the radiation budget (Ponater et al., 1996). The impact of contrails
on transmission of radiation depends on their optical depth. In the solar range
(near a wavelength of 0.55 µm), the optical depth of observed persistent contrails
varies typically between 0.1 and 0.5 (Kästner et al., 1993; Sassen, 1997; Jäger
et al., 1998; Minnis et al., 1998a). Occasionally, very thick contrails (on
the order of 700 m) with optical depths greater than 1.0 are found at higher
temperatures (up to -30°C) (Schumann and Wendling, 1990; Gayet et al., 1996).
The optical depth in the 10-µm range is about half that near 0.55 µm (Duda and
Spinhirne, 1996). Particles in young persistent contrails are typically smaller
(mean diameter 10 to 30 µm) than in other cirrus cloud types (greater than 30
µm) (Brogniez et al., 1995; Gayet et al., 1996) but grow as the contrail ages
and may approach the size of natural cirrus particles within a time scale on
the order of 1 h (see Section 3.4) (Minnis et al., 1998a).
The number density of ice crystals in contrails (on the order of 10 to 200 cm-3)
is much larger than in cirrus clouds (Sassen, 1997; Schröder et al., 1998b).
Reported IWC values in aged contrails vary between 0.7 and 18 mg m-3 (Gayet
et al., 1996; Schröder et al., 1998b), consistent with results from numerical
studies (Gierens, 1996). As for cirrus clouds (Heymsfield, 1993; Heymsfield
et al., 1998b), the IWC of contrails is expected to depend on ambient temperature
(Meerkötter et al., 1999) because the amount of water mass available between
liquid and ice saturation (for temperatures < -12°C) increases with temperature
(Ludlam, 1980). Hence, contrails may be optically thicker at lower altitudes
and higher temperatures. Contrail particles have been found to contain soot
(see Section 3.2.3). Soot in or on ice particles may increase
absorption of solar radiation by the ice particles, hence reduce the albedo
of the contrails. The importance of soot depends on the type of internal or
external mixing and the volume fraction of soot enclosures. Because soot particles
are typically less than 1 mm in diameter, their impact on the optical properties
of ice particles in aged contrails is likely to be small. Contrail particles
often deviate from a spherical shape (see Section 3.4.4).
The magnitude and possibly even the sign of the mean net radiative forcing of
contrails depends on the diurnal cycle of contrail cover. For the same contrail
cover, the net radiative forcing is larger at night. Satellite data reveal a
day/night contrail cover ratio of about 2 to 3 (Bakan et al., 1994; Mannstein,
1997). Aviation fuel consumption inventories suggest a longitude-dependent noon/midnight
contrail cover ratio of 2.8 as a global mean value (Schmitt and Brunner, 1997).
Table 3-8: Shortwave (SW), longwave (LW), and net radiative
flux changes (Wm-2) at top of atmospherein global mean as caused by contrails
in 1992 and 2050 scenario Fa1 (see Section 3.7.2
and Chapter 9), for contrail cover as shown in Figure
3-16 and for solar optical depths of 0.1, 0.3, and 0.5 for contrails
(Minnis et al., 1999). The last line gives the results for temperature-dependent
ice water content (IWC) with variable optical depth.
|
|
Optical Depth at 0.55 µm |
SW
|
LW
|
Net
|
SW
|
LW
|
Net
|
|
|
|
|
|
|
|
0.1 |
-0.0030
|
0.0111
|
0.0081
|
-0.018
|
0.067
|
0.049
|
0.3 |
-0.0081
|
0.0246
|
0.0165
|
-0.049
|
0.148
|
0.099
|
0.5 |
-0.0124
|
0.0327
|
0.0203
|
-0.075
|
0.197
|
0.122
|
Variable (from temperature-dependent IWC) |
-0.0038
|
0.0135
|
0.0097
|
-0.024
|
0.084
|
0.060
|
|
|
|
a) Cloud amount, cloud distribution, and cloud optical depth
are 1986 ISCCP 3-h data interpolated to 1 h, for 4 months (January, April,
June, October), with mean cloud cover of 68% of the Earth surface.Ice particles
are modeled as hexagons with mean diameters of 20 µm. Water clouds consist
of droplets with 60-µm mean diameter. Surface skin temperature and surface
albedo are taken from ISCCP and Staylor and Wilber (1990). Winter and standard
temperature and moisture profiles are assumed for pressures < 50 hPa, and
numerical weather analysis monthly mean profiles of National Meteorological
Center (NMC; now National Center for Environmental Protection, NCEP) are
used for pressures > 50 hPa. Continental and marine aerosols were also included
in the model. Contrails are assigned at a pressure
of 200 hPa and assumed tobe 220-m thick with aspherical ice particles of
24-µm volume mean diameter.IWC in contrails was adjusted to result in anoptical
depth of 0.1, 0.3, or 0.5. A case with IWC set to half the amount of water
available for ice formation from vapor at 100% humidity relative to liquid
saturation (temperature-dependent IWC) is also considered (variable optical
depth).Net TOA forcing is computed with model FL as difference of results
with and without contrails. |
|
3.6.4. Radiative Forcing of Line-Shaped Contrail Cirrus
Model studies indicate the importance of contrails in changing the Earth's
radiation budget. One-dimensional (1-D) models represent contrails as plane-parallel
clouds in a homogeneously layered atmosphere and use area-weighted sums for
fractional contrail cover (Fortuin et al., 1995; Strauss et al., 1997; Meerkötter
et al., 1999). As a consequence, contrail forcing grows linearly with contrail
cover in these models. Inhomogeneity effects may be large in natural cirrus
(Kinne et al., 1997) and small for vertically thin contrail clouds (Schulz,
1998) but may be important for thick and narrow contrails. Computations of radiative
forcing by contrails have been done for fixed atmospheric temperatures in the
North Atlantic flight corridor (Fortuin et al., 1995). Normalized to 100% contrail
cover (as provided by 1-D models), these computations found a net forcing in
the range of -30 to +60 W m-2 in summer and 10 to 60 W m-2 in winter. The negative
forcing values apply to clouds that are much thicker than typical contrails.
A radiative convective model used to simulate the climatic conditions of a mid-European
region (Strauss et al., 1997) found a radiative flux change of almost 30 W m-2
at the tropopause for 100% contrail cover with 0.55-µm optical depth of 0.28,
and a surface temperature increase on the order of 0.05 K for a 0.5% increase
in current contrail cloud cover. With a 2-D radiative convective model, a 1
K increase was found in surface temperature over most of the Northern Hemisphere
for an additional cirrus cover of 5% (Liou et al., 1990). The potential effects
of contrails on global climate were simulated with a GCM that introduced additional
cirrus cover with the same optical properties as natural cirrus in air traffic
regions with large fuel consumption (Ponater et al., 1996). The induced temperature
change was more than 1 K at the Earth's surface in Northern mid-latitudes for
5% additional cirrus cloud cover in the main traffic regions. Meerkötter et
al. (1998) applied three established radiation transfer models to compute the
static radiative forcing due to a prescribed additional cloud cover by contrails.
These models, which assume plane parallel cloud cover, are the two- and four-stream
models of Fu and Liou (1993) (FL); the matrix operator method of Plass et al.
(1973), also used by Strauss et al. (1997) (M); and the four-stream model of
Nakajima and Tanaka (1986, 1988) (N). The FL and M models have participated
in model comparison exercises (Ellingson and Fouquart, 1990). Table
3-6 on the previous page shows the results of a parameter study that was
carried out for various regions and seasons using models M and FL for spherical
and hexagonal particles. The contrails cause a net forcing that is positive
in all cases after summing over negative SW and positive
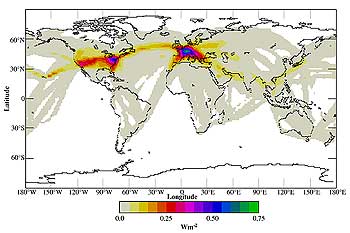
Figure 3-21: Global distribution of net instantaneous radiative forcing
at the top of atmosphere in daily and annual average for present (1992) climatic
conditions and analyzed contrail cover (see Figure 3-16) and 0.55-µm optical depth of
0.3 (Minnis et al., 1999).
|
LW flux changes. The magnitude of SW forcing is larger over dark ocean than
over bright snow surfaces and larger for hexagonal ice particles than for spheres.
LW forcing is larger in the tropics than in polar regions. Despite the small
ocean albedo, net forcing is largest in the tropics because it has the warmest
lower atmosphere. These results are consistent with the expectation that net
forcing is smallest over cool and dark surfaces. Net forcing over the mid-latitude
continent is larger in summer than in winter. Hexagonal particles cause a larger
albedo than spherical ones, therefore less net forcing. These results show that
contrail heating generally prevails over cooling in the atmosphere-surface system.
However, this finding does not preclude situations in which contrails cause
a net cooling-for example, very cold surface, high atmospheric humidity, low
surface albedo, very small particles (< 10 µm; the limit depends on the ice
water path in the cloud), or large optical contrail depth (> 10). TOA radiative
forcing depends mainly on the cover and on parameters that determine the solar
optical depth of contrails and to a minor degree on other parameters. Forcing
is very weakly sensitive to the methods used for radiative transfer calculations.
This sensitivity can be seen from Table 3-7, which
lists the results of a parameter sensitivity study with models FL, M, and N
(Meerkötter et al., 1999). Except for the first two rows of Table
3-7, results are from model N only because the results of the three models
agree with each other to within 3%. The largest model differences are found
for aspherical particles, which are represented by different shapes in various
spectral regions in the models, but all models show smaller net forcing for
aspherical particles than for spherical ones, as expected (Kinne and Liou, 1989).
The results also depend rather strongly on solar zenith angle, ice water content,
particle diameter, surface albedo, and relative humidity. Low-level clouds with
large cover and optical depth reduce SW and LW forcing of the cloud-free case
below a contrail, causing a small net increase of forcing. Variations in surface
temperature (here ± 5 K) cause small LW flux changes. Lowering the altitude
of a contrail for fixed IWC reduces the LW effect slightly. Lowering the altitude
of the contrail and using the IWC that is expected for the higher temperature
at lower levels makes the lower contrail optically thicker and radiatively more
effective than the higher contrail. Hence, TOA radiative forcing by contrails
grows with increasing surface temperature, surface albedo, and IWC. For the
same IWC, contrails with small ice particles are more effective in radiative
forcing than contrails with larger particles. Representative forcing values
are approximately 25 to 40 W m-2 for 100% contrail cover and 0.55-µm optical
depth of 0.5. Radiative forcing by contrails depends strongly on the optical
depth of the contrails and is different at the surface than at the TOA. Figure
3-20 shows computed SW, LW, and net change in radiative fluxes at the TOA
(actually 50 km) and at the surface for 100% contrail cloud cover for the mid-latitude
summer continental reference case with spherical ice particles. The ice water
content was varied to yield different values of the 0.55-µm optical depth t.
The trends are consistent with those found in cirrus studies (Fu and Liou, 1993).
At the TOA, LW forcing is larger than SW forcing, giving a net heating of the
atmosphere that is maximum near t = 3. The flux increases slightly less than
linearly with optical depth for small values of t. The net forcing changes sign
and becomes negative for t > 10 (not shown), but contrails are probably never
that optically thick. This analysis indicates that contrails heat the atmosphere
below them.
Table 3-9: Global radiative forcing by contrails and indirect
cloud effects in 1992 and 2050 (scenario Fa1). No entry indicates insufficient
information for best-estimate value.
|
Radiative Forcing |
Best Estimate
or Range
|
Uncertainty Range
with 2/3 Probability
|
Status of
Understanding
|
1992 |
|
|
|
Line-shaped contrail cirrus |
0.02 Wm-2
|
0.005-0.06 Wm-2
|
fair
|
Additional aviation-induced cirrus clouds |
0-0.04 Wm-2
|
-
|
very poor
|
Other indirect cloud effects |
-
|
either sign, unknown magnitude
|
very poor
|
|
|
|
|
2050 |
|
|
|
Line-shaped contrail cirrus |
0.10 Wm-2
|
0.03-0.4 Wm-2
|
fair
|
Additional aviation-induced cirrus clouds |
0-0.16 Wm-2
|
-
|
very poor
|
Other indirect cloud effects |
-
|
either sign, unknown range
|
very poor
|
|
|
In all cases, TOA and top of the troposphere radiative flux changes from contrails
differ by only about 10%; therefore, the instantaneous or static flux change
gives a reasonable approximation for the adjusted radiative forcing as considered
in IPCC (1996).
At the Earth's surface, LW flux changes are much smaller because water vapor
closes much of the infrared radiation window in the lower atmosphere. In contrast,
SW flux changes are only a little smaller than at TOA. Hence, the daytime SW
contribution dominates and cools the surface in the daily mean. A reduction
of solar radiation by 40 W m-2 has been measured locally in the shadow of contrails,
although the simultaneous change in infrared flux in the shadow of contrails
was very small (Sassen, 1997). Hence, the Earth's surface is locally cooled
in the shadow of contrails. This analysis does not exclude warming of the entire
atmosphere-surface system driven by the net flux change at TOA. As radiation-convection
models show, for example, vertical heat exchange in the atmosphere may cause
a warming of the surface even when it receives less energy by radiation (Strauss
et al., 1997). Cooling of the atmosphere below contrails is also suggested by
measurements of solar and infrared upward and downward fluxes above and below
a few persistent contrails (Kuhn, 1970). These measurements show a strong (10-20%)
reduction of net downward radiation just below approximately 500-m-thick contrails
with little change in LW fluxes. Further investigation is required to demonstrate
how these results depend on the geometry and age of contrails.
Contrail cirrus induce a heat source by the change in divergence of solar and
infrared radiation fluxes mainly within but also below the contrail in the upper
troposphere (Liou et al., 1990; Strauss et al., 1997; Meerkötter et al., 1999).
In the atmosphere below a contrail, the change in heat source is on the order
of 0.3 K/day for 100% cover. When the contrail is located above a thick lower
level cloud, the atmosphere is heated only above the lower cloud; the heat source
is essentially zero below the lower cloud. In the diurnal cycle, radiative forcing
by contrails is positive and strongest during the night because of the absence
of negative SW forcing (see Table 3-6). For small
optical depth, net forcing at TOA is also positive during the day, hence always
positive regardless of the diurnal cycle of contrail cover. Negative SW forcing
is maximum not at noon but during morning or afternoon hours, when the solar
zenith angle is near 70°. Other than at TOA, the net flux change is negative
at the surface even for thin contrails during the day, in particular at intermediate
zenith angles. The maximum day-night difference in net radiative forcing at
the surface for cloudless summer mid-latitude conditions is about 20 W m-2 for
constant 100% contrail cover with optical depth of 1 and constant surface temperature,
and slightly less when accounting for the daily temperature cycle.
Table 3-10: Parameters affecting future changes in aircraft-produced
aerosols and cloudiness and theirimpacts on contrails and aerosol abundance.
Symbols indicate sign of change in the parameter and in the impact. Question
marks indicate uncertainty in sign or importance of an impact.
The symbol x indicates lack of a known or important impact.
|
Parameter
|
Sign of
Change
|
Global Contrail
and Induced-
Cirrus Coverage
|
Global Contrail
Radiative
Forcing
|
Aerosol
Abundance
|
|
|
|
|
|
Upper troposphere temperature |
+? |
- |
- |
x |
Lower stratosphere temperature |
- |
x |
x |
+ |
Humidity of lower stratosphere |
+ |
x |
x |
+ |
Humidity of upper troposphere |
+? |
+ |
+ |
+ |
Tropopause altitude |
+ |
+ |
+ |
x |
Number of aircraft |
+ |
+ |
+ |
+ |
Global aviation fuel consumption |
+ |
+ |
+ |
+ |
Overall efficiency of propulsion |
+ |
+ |
+ |
x |
Cruise altitude at mid-latitudes |
+ |
- |
- |
+ |
Cruise altitude in the tropics |
+ |
+ |
+ |
x |
Traffic in tropical regions |
+ |
+ |
+ |
x |
Soot emissions |
-? |
-? |
-? |
x |
Fuel sulfur content |
-? |
-? |
-? |
- |
Fuels with higher hydrogen content |
+? |
+ |
? |
- |
|
|
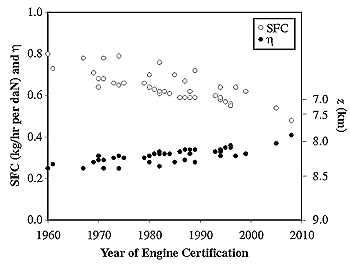
Figure 3-22: Trend in overall efficiency of propulsion h (solid
circles), computed from aircraft specific fuel consumption (SFC) data
(open circles; data as in Figure 7-9), according
to h = V(Q SFC)-1, with V as the aircraft speed (~240 m s-1 ) and Q as
the specific heat of combustion of aviation fuels (43 MJ kg-1). Solid
circles also denote the critical altitude z (right axis) above which contrails
form (for 100% relative humidity in the mid-latitude standard atmosphere)
for the years 1960 to 2010.
|
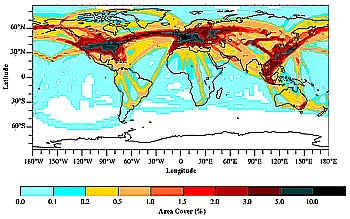
Figure 3-23: Persistent contrail coverage (in % area cover) based on
meteorological analysis data and on fuel emission database for 2050 (Fa1 fuel
consumption scenario for 2050), assuming linear dependence on fuel consumption and
overall efficiency of propulsion h of 0.5; global mean cover is 0.5%. Compare with
Figure 3-16 (from Gierens et al., 1998).
|
The global distribution of radiative forcing from contrails can be estimated
using a radiation transfer model, the expected contrail cover, and a realistic
representation of the cloudy atmosphere and surface (Minnis et al., 1999). Global
contrail cover for 1992, for example, is shown in Figure
3-16 (see Section 3.4.3). Global mean values for
TOA radiative flux are summarized in Table 3-8,
along with details of the calculations. As expected, SW forcing is negative
and LW and net forcings are positive. All three values increase less than linearly
with solar optical depth t. The resulting 1992 global distribution of TOA net
forcing for t of 0.3 is shown in Figure 3-21. Forcing
is largest in regions of heavy air traffic, with maximum values over northeast
France (0.71 W m-2) and near New York (0.58 W m-2). Although the contrail amount
is higher over the northeast United States of America, net contrail forcing
over Europe is greater because of the greater LW forcing term. The global mean
net forcing for t between 0.3 and 0.5 is about 0.02 W m-2; the zonal mean value
is largest near 40°N, with a value five times larger than the global mean.
Computed results for global radiative forcing by contrails depend on assumed
values for contrail cover and mean optical depth of contrails. Neither is well
known. Here, the computed global contrail cover was normalized to yield 0.5%
observed cover by line-shaped contrails over Europe, guided by satellite data.
Because satellite data mainly reveal thicker contrails, a larger cover resulting
from the presence of optically thin contrail cirrus might not be accurately
detected. Therefore, the results for larger t values (0.3 to 0.5) are considered
in combination with the given contrail cover (0.1% globally) as being most representative
for real forcing conditions. Hence, in the diurnal and annual mean, a global
0.1% increase in thin contrail cloud cover causes a net heating of the Earth-atmosphere
system of approximately 0.02 W m-2. The difference between the largest and smallest
net forcing values in Table 3-8 suggests an error
bound on the order of 0.01 W m-2. Based on Section 3.5,
the actual global cover may (with 2/3 probability) be 2 to 3 times smaller or
larger than the derived line-shaped contrail cover. The optical depth value
is likely known to a factor of 2 to 3. Thus, for assumed Gaussian behavior of
individual uncertainties, the radiative forcing value may differ from the best
estimate by a factor of about 3 to 4.
Based on computations using estimates of line-shaped contrail occurrence for
the 1992 fuel scenario (0.1% cover), the best estimate of global-mean radiative
forcing is positive, has a value of about 0.02 W m-2 with an uncertainty factor
of 3 to 4, and may range from 0.005 to 0.06 W m-2 for present climate conditions.
Certainly, the state of our understanding is only fair. Future investigations
may result in considerable changes to the best estimates.
Global radiative forcing by contrails obviously is much smaller than that attributed
to other anthropogenic changes in the past century-1.5 W m-2, which
represents about the median of the range of values given in IPCC (1996) but
comparable to the forcing by past CO2 emissions
by aircraft (Brasseur et al., 1998; see Chapter 6). A
mean radiative forcing of 0.02 W m-2 induces a vertically averaged
heat source in the troposphere equivalent to approximately 0.0002 K day-1. The
atmosphere reacts to this heat source in a complex manner (Ponater et al., 1996)
and may take decades to reach a steady-state temperature response. In steady
state, the mean surface temperature may increase by about 0.01 to 0.02 K globally
if climate sensitivity from contrail forcing is comparable to that of well-mixed
greenhouse gases (IPCC, 1996; see Section 6.2.1).
In comparison to the global mean value, annually averaged zonal mean values
are larger by a factor of about 5, and regional values are larger by a factor
of up to 40 (see Figure 3-21). The pattern of the
climate response differs from the pattern of radiative forcing, in particular
at small regional scales. Zonal mean steady-state temperature changes of between
0.01 and 0.1 K appear to be possible for present contrail cover when previous
studies as scaled to the cover and radiative forcing found here (Liou et al.,
1990; Ponater et al., 1996; Strauss et al., 1997). Regional contrail forcing
may have short-term consequences for the daily temperature range in such a region
if contrail forcing persists for at least a day. These temperature changes appear
to be too small to be detectable in comparison to atmospheric temperature variations.
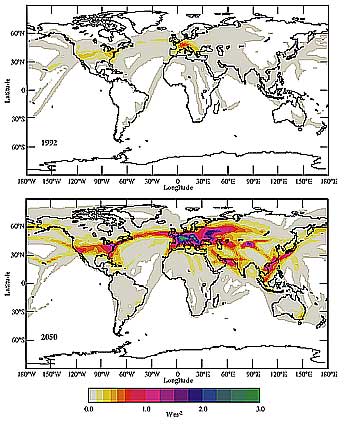
Figure 3-24: Global distribution of net radiative forcing at top
of atmosphere in daily and annual average for contrails with 0.55-µm optical depth
of 0.3: For 1992, as in Figure 3-21; and for the 2050 scenario with contrail cover
as shown in Figure 3-23 (Minnis et al., 1999).
|
3.6.5. Radiative Impact of Additional or Changed Cirrus
and Other Indirect Effects
Besides forcing by line-shaped contrail cirrus, the global radiation balance
may also be perturbed if there is a significant indirect effect of aircraft-induced
aerosol, water vapor, and contrails on the coverage and properties (particle
size distribution, number density, and composition) of "natural" cirrus clouds.
In addition, there might be other indirect cloud-related effects, such as changes
in humidity and precipitation. The principal effects might be similar to those
caused by volcanic aerosol mixed down into the troposphere (see Section
3.4.5).
Radiative forcing will be enhanced by any increase in the cover of thin cirrus
caused by aircraft beyond that of line-shaped contrail cirrus. If the additional
global cirrus cover is as large as 0.2% (the estimated upper bound for 1992;
see Section 3.5.1.5), and if the optical properties
of this additional cirrus are the same as for line-shaped persistent contrails
(optical depth of about 0.3, as also found often for natural cirrus; see Section
3.4.1), then the radiative forcing from the additional cirrus may be as
large as 0.04 W m-2-which is twice the value for line-shaped contrail cirrus.
Aircraft emissions may also change the properties of natural cirrus clouds
(see Section 3.4.5). In a high-traffic region, cirrus
was found to be affected by soot emissions from aircraft, causing an approximate
doubling of the ice particle concentration (Ström and Ohlsson, 1998). Smaller
particles cause larger optical depth for constant ice water content. Radiative
forcing is strongly sensitive to particle size (see Table
3-7). As Figure 3-20 indicates, an increase in
optical depth causes additional heating if the cirrus cloud was optically thin
but cooling if it was optically thick (Wyser and Ström, 1998). One recent study
suggests that the indirect heating effect of aviation-induced changes in cirrus
ice particle number density for fixed cloud cover may be positive and comparable
to or even larger than that from increases in cloud cover (Meerkötter et al.,
1999).
While the impact of particle changes on radiative forcing by cirrus clouds
may be studied parametrically, our understanding is very poor with respect to
other indirect effects. Although comprehensive investigations are missing, there
is no evidence that any of the indirect effects are important. Table
3-9 summarizes the assessment of global radiative forcing by aviation-induced
cloudiness for 1992. The results for 2050 are explained in Section
3.7.
|